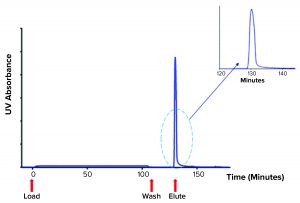
Figure 1: Chromatogram showing platelet releasate impurities being washed from a ligand-based exosome affinity purification (LEAP) column, before a buffer change eluted the purified EVs from the column
Novel therapeutics based on extracellular vesicles (EVs) recently passed a critical development milestone. During 2020, some of the first experimental EV products developed by biopharmaceutical companies entered human clinical trials (1–3). EVs are nanometer-sized, lipid-wrapped spheres released by almost every cell type in the human body. EVs are loaded with a cargo of proteins, lipids, and RNA, and they are tagged with surface markers that favor uptake by target cells. Thus, EVs are a key mode of cell-to-cell communication (4). As such, they offer intriguing potential to be used therapeutically.
Naive EVs (NEVs) isolated from sources such as platelets and stem cells are of great interest in regenerative medicine. Possible applications of NEVs include wound healing and osteoarthritis treatment (5). The potential of modified EVs (e.g., “engineered EVs” or EEVs) to act as targeted, highly biocompatible, nonimmunogenic drug delivery vehicles already has captured the attention of some big pharmaceutical companies (6).
As EV therapeutics start to transition through clinical trials, however, a major development bottleneck looms. Although bioreactor-cultured cells produce trillions of EVs (upstream processing stage), the downstream processing (purification) of EVs traditionally has been based on ultracentrifugation (UC). Studies have acknowledged that UC protocols are labor-intensive, technically demanding, slow, and not directly scalable (7–9). Concerns also have been raised over the integrity and purity of EVs processed with UC, and the levels of contamination remaining in final products (10). Across related industries, including viral and vaccine production, UC has been phased out wherever possible (9).
Although NEV products might not need to meet stringent purity requirements, EEVs are designed to harness unique characteristics of natural and modified EVs to deliver a “cargo” of drugs. Thus, product purity and consistency are important for those drug delivery vehicles.
For EEV production to achieve commercial viability, an alternative downstream processing method to UC might be needed. Of the methods that have been explored, size-exclusion chromatography and microfluidics-based (particle-size) approaches lack scalability and output purity because aggregates of proteins or other cellular materials of similar size to EVs can be coisolated in the filtrate. Polymer-based exosome precipitation also can be difficult to scale, does not meet purity requirements, and can cause polymer contamination (11). By contrast, tangential-flow filtration (TFF) is scalable, but it can result in low product purity (high contamination) when used alone (12).
Typically, combining processes leads to poor yields and higher processing times. Immunoaffinity-based EV capture, based on the recognition of specific markers on an EV population’s surface, might find its niche in EV-based diagnostics. But immunoaffinity-based EV capture does not offer a general platform for EV purification, and it is expected to be cost-prohibitive at manufacturing scales (11).
In neighboring areas of biopharmaceutical production, including protein and mRNA manufacturing, ion-exchange chromatography (IEX) plays a key role in downstream processing (13). Below, we describe a proprietary IEX-based protocol specifically designed and validated for EV isolation and purification. It is suitable for a broad range of upstream sources and can be used with standard bioprocessing equipment at any scale from bench to commercial manufacturing. In our studies, ligand-based exosome affinity purification (LEAP) technology has proven to be an efficient, scalable, low-cost, and highly reproducible EV purification platform technology suited to the manufacture of NEV and EEV products.
LEAP Technology
The LEAP platform is based on selective capture (binding) and ready release (elution) of EVs from EV-containing media (e.g., cell culture media, activated platelet releasate). Previous research into EV IEX primarily has explored anion-exchange chromatography (AEX) for EV purification (14, 15). By contrast, LEAP technology is based on cation exchange. Although EVs have a net-negative charge, local areas of positive charge exist on the outer surface of their lipid bilayer structure. LEAP technology uses charged R groups spaced about 5 Å apart along the LEAP resin “backbone” to selectively capture EVs by interacting with those positive charges (16). LEAP intellectual property is based on the discovery that there are many interchangeable, specifically charged R groups and backbone chemistries applicable to EV purification.
The LEAP purification protocol is based on scalable unit operations such as filtration and column chromatography. It uses materials and techniques already implemented routinely for processing monoclonal antibodies (MAbs) and vaccines. The platform should be capable of routine purification from bench scale to commercial manufacturing scale of 2,000 L using only industry-standard equipment that most contract manufacturing organizations have on hand.
After each run, LEAP resins can be sanitized and reused repeatedly, without significant loss in binding capacity. That reduces the cost per use for EV manufacturing. LEAP ligand groups are chemically stable and have no risk of denaturation, misfolding, or other ligand-leaching issues that are common in protein-based immunoaffinity chromatography.
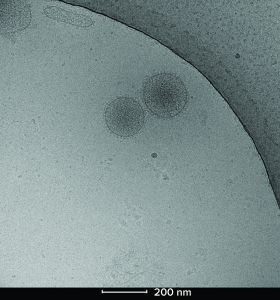
Figure 2: Cryoelectron microscopy image of two Plexaris platelet-derived extracellular vesicles isolated using ligand-based exosome affinity purification (LEAP) technology; the image shows the characteristic lipid bilayer, dense core, and integral proteins observed as spikes on the surface.
Case Study:
Platelet-Derived EV Isolation
Our goal was to demonstrate the suitability of the LEAP platform for scalable downstream EV processing of a clinical-grade product. We used the technology to manufacture platelet-derived EVs (Plexaris from Exopharm Limited) as potential therapeutics for enhanced wound healing. To date, this program has proceeded to full patient dosing in two phase 1 clinical trials for safety and wound healing (1, 2).
To produce platelet-derived EVs for clinical study, we activated platelets from pooled packs sourced from a clinical-grade platelet supplier. We used our in-house method to stimulate EV release and processed the resulting releasate through the LEAP platform. After an initial filtration step, we loaded releasate onto a column packed with the LEAP resin. A washing step removed the bulk of free protein, including histones, particles, and other impurities in the releasate. Once those impurities had been washed from the column, a simple change of buffer readily eluted the purified EVs in a concentrated burst (Figure 1).
For Plexaris EVs, the LEAP platform provided about 70× concentration factor. Depending on the dilution of the EV source, LEAP technology can provide up to a 200× concentration factor, thus simplifying subsequent ultrafiltration and sterile filtration steps involved in formulation, fill, and finish. The platform isolated and recovered a consistent high yield of platelet EVs (pEVs) from the activated platelet packs, as shown by both protein quantity and particle count measurements over 10 runs (Table 1).
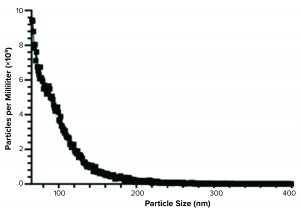
Figure 3: Spectradyne particle analysis of Plexaris platelet-derived extracellular vesicles (EVs) isolated using ligand-based exosome affinity purification (LEAP) technology
Characterization of pEVs
Following the isolation step, we characterized Plexaris EVs to establish key biophysical and biochemical properties of the captured EVs. Our goal was to confirm the LEAP platform’s capability to manufacture EVs at high quantity and quality and in compliance with guidelines applicable to EVs (17).
We applied different complementary techniques to validate the characteristics of Plexaris EVs isolated by the LEAP platform. Analysis of the isolated pEVs using negative staining transmission electron microscopy (TEM) revealed spherical cup-like particles. That result was consistent with other observations of EV morphology (17).
We used cryo-TEM to visualize the particle distribution and shape of the pEVs (Figure 2). The particles exhibited the expected bilayer structure, solid core, and protein attachments. Those key characteristics match what has been previously published for similar EVs. Plexaris EVs were a heterogeneous population that contains small, medium, and large EVs with sizes ranging from 30 nm to >200 nm.
We confirmed electron microscopy results using the nCS1 particle measuring instrument from Spectradyne. Mean resistive pulse sensing can detect particles between 65 nm and 400 nm with the TS-400 chipset. The data generated from that instrument (Figure 3) conformed with the data from the electron microscopy analysis.
Platelet EV protein biomarkers have been well characterized (18). We used a microfluidic Western blot system from ProteinSimple (Jess) to characterize the LEAP-purified pEVs. That system offers several advantages over traditional Western blotting. They include the capability to probe multiple targets from a sample simultaneously and to obtain accurate molecular weight measurements. The platelet EV protein biomarkers detected — including CD41, CD9, and annexin V (Figure 4) — confirm that Plexaris LEAP-purified product contains EVs.
Batches of LEAP-derived pEVs contained a typical protein-to-particle ratio of 0.167 µg protein per 108 particles (Table 1). By comparison, Aatonen et al. reported that pEVs recovered by UC had a protein-to-particle ratio of 3.42–29.97 µg protein per 108 particles (19). That comparison suggests that LEAP yielded purer pEV isolates, with up to 20–180 times fewer contaminant proteins than could be obtained by using UC.
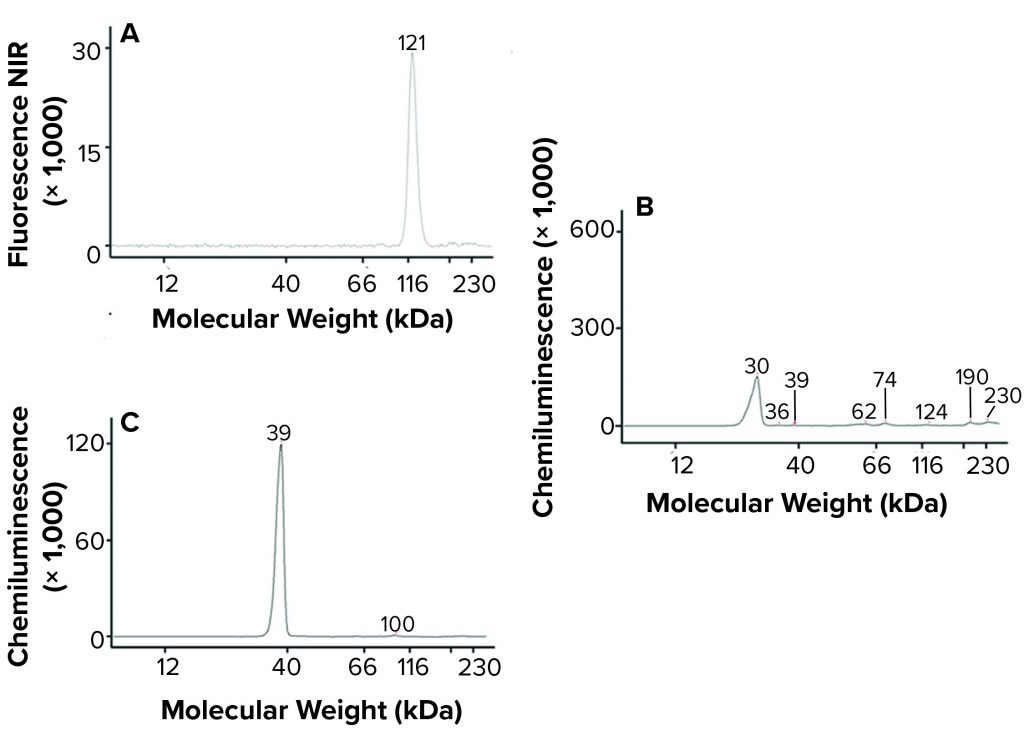
Figure 4: Jess microfluidic Western analysis of EV markers on Plexaris platelet-derived extracellular vesicles (EVs) isolated by ligand-based exosome affinity purification (LEAP) technology; (a) CD41, (b) CD9, (c) Annexin V; note that relative peak height is not indicative of abundance. (NIR = near infrared)
Scalability
During the initial research phase of Plexaris EV development, downstream processing typically was carried out using sub-400-mL pooled packs of platelets. To generate sufficient clinical-grade material for clinical trials, the LEAP process was scaled up. At current process scales, the unit operation required two operators working about eight hours to produce 40 doses.
We expect that further scale-up of the technology will not increase process time significantly. With standard automation of fill–finish, larger scale production would take less time than required for the small-scale manual filling processes for 40 doses. Producing 300 doses would require larger, commercially available equipment (Table 2). Vial filling would be performed using additional mechanized systems, so processing time would decrease further.
Producing 2,000 or 12,000 doses simply would require yet larger industry-standard equipment and semiautomated vial fill and finish systems. So the time to completion would not be expected to change.
By contrast, using UC to scale from 4 L of media to 400 L would take 500 times longer and require about 100 times more labor than the LEAP method. We believe that LEAP is readily scalable to over 2,000 L using industry-standard equipment and processes.
Clearing Bottlenecks
Selection of a purification method to isolate EVs for commercial therapeutic application requires careful consideration of cost, reproducibility, and yield balanced against purity and quality needs (Table 3). Methods that achieve low yield such as UC or microfluidics are unlikely to meet the needs of commercial manufacturing. Methods that require expensive equipment or reagents (e.g., UC, commercial precipitation kits, or immunoaffinity) present significant challenges that might be insurmountable at commercial scales (7–9).
The LEAP platform has proven to be a robust and reliable, low-cost, high-yield downstream process for clinical-grade EV manufacture, which should be highly amenable to scale-up using only industry-standard equipment. Compared with other modes of EV purification, this downstream processing platform offers a combination of advantages and benefits that can remove the EV manufacturing bottleneck. That enables EV-based therapeutics to continue to progress into later stage clinical trials —ultimately reaching their full clinical potential.
EV medicines could be used to treat many different medical problems, and small-scale clinical trials are now underway. Potential variants of EV medicines are possible, but downstream processing difficulties are the biggest obstacles in the pathway toward registration studies and commercial use. The ligand-based exosome affinity purification technology described above and the supporting data provided point to a proprietary answer to the downstream processing bottleneck.
References
1 A Phase I Clinical Study to Evaluate Safety, Tolerability, and Biological Activity of Platelet-Derived Extracellular Vesicles on Wound Healing in Healthy Adults. Australian New Zealand Clinical Trials Registry, ACTRN12619001378112; http://anzctr.org.au/Trial/Registration/TrialReview.aspx?id=378212.
2 Phase I Study to Assess the Safety of a Human Non-Autologous Platelet Derived Extracellular Vesicle Therapy in Wound Healing. Australian New Zealand Clinical Trials. Registry, ACTRN12620000944932; http://anzctr.org.au/Trial/Registration/TrialReview.aspx?id=380050.
3 A First-in-Human Study of CDK-002 (exoSTING) in Subjects With Advanced/Metastatic, Recurrent, Injectable Solid Tumors. US Clinical Trial NCT04592484; https://clinicaltrials.gov/ct2/show/NCT04592484.
4 Kalluri R, Le Bleu VS. The Biology, Function and Biomedical Applications of Exosomes. Science 367, 2020: eaau6977; https://doi.org/10.1126/science.aau6977.
5 Wiklander OPB, et al. Advances in Therapeutic Applications of Extracellular Vesicles. Sci. Transl. Med. 11(492) 2019: eaav8521; https://doi.org/10.1126/scitranslmed.aav8521.
6 Zipkin M. Big Pharma Buys into Exosomes for Drug Delivery. Nat. Biotechnol. 38, 2020: 1226–1228; https://doi.org/10.1038/s41587-020-0725-7.
7 Furi I, Momen-Heravi F, Szabo G. Extracellular Vesicle Isolation: Present and Future. Ann. Transl. Med. 5(12) 2017: 263–263; https://doi.org/10.21037/atm.2017.03.95.
8 Konoshenko MY, et al. Isolation of Extracellular Vesicles: General Methodologies and Latest Trends. BioMed. Res. Int. 2018, 2018: 8545347; https://doi.org/10.1155/2018/8545347.
9 Colao IL, et al. Manufacturing Exosomes: A Promising Therapeutic Platform. Trends Mol. Med. 24(3) 2018: 242–256; https://doi.org/10.1016/j.molmed.2018.01.006.
10 Forteza-Genestra MA, et al. Purity Determines the Effect of Extracellular Vesicles Derived from Mesenchymal Stromal Cells. Cells 9(2) 2020: 422; https://doi.org/10.3390/cells9020422.
11 Li P, et al. Progress in Exosome Isolation Techniques. Theranostics 7(3) 2017: 789–804; https://doi.org/10.7150/thno.18133.
12 Nordin JZ, et al. Ultrafiltration with Size-Exclusion Liquid Chromatography for High Yield Isolation of Extracellular Vesicles Preserving Intact Biophysical and Functional Properties. Nanomedicine 11(4) 2015: 879–883; https://doi.org/10.1016/j.nano.2015.01.003.
13 Scott C. Ion-Exchange Chromatography for Modern Biopharmaceutical Purification. BioProcess Int. eBook, 18 December 2020; http://lne.e92.mwp.accessdomain.com/downstream-processing/chromatography/ebook-iex-chromatography-for-modern-biopharmaceutical-purification.
14 Heath N, et al. Rapid Isolation and Enrichment of Extracellular Vesicle Preparations Using Anion Exchange Chromatography. Sci. Rep. 8(1) 2018: 5730; https://doi.org/10.1038/s41598-018-24163-y.
15 Staubach S, et al. Challenges in Industrial Process Development of Exosome-Based Therapies: Characterizing and Managing Diversity. BioProcess Int. eBook,
25 August 2020; http://lne.e92.mwp.accessdomain.com/analytical/downstream-development/classification-of-extracellular-vesicles-using-chromatography-for-exosome-therapy-production.
16 Joseph C, et al. Methods and Compositions for Purification or Isolation of Microvesicles and Exosomes. International Patent WO2018112557A1, 28 June 2018.
17 Théry C, et al. Minimal Information for Studies of Extracellular Vesicles: (MISEV2018)AS — Position Statement of the International Society for Extracellular Vesicles and Update of the MISEV2014 Guidelines. J. Extracell. Ves. 7(1) 2018: 1535750; https://doi.org/10.1080/20013078.2018.1535750.
18 Johnson J, et al. Prospective Therapeutic Applications of Platelet Extracellular Vesicles. Trends Biotechnol. 4 November 2020; https://doi.org/10.1016/j.tibtech.2020.10.004.
19 Aatonen MT, et al. Isolation and Characterization of Platelet-Derived Extracellular Vesicles. J. Extracell. Ves. 3(1) 2014: 24692; https://doi.org/10.3402/jev.v3.24692.
Corresponding author Sam Law is process engineer at Exopharm Ltd., Level 17, 31 Queen Street, Melbourne, VIC 3000, Australia. Jancy Johnson is a research assistant at Exopharm Ltd. and PhD student at the Department of Biochemistry and Pharmacology at the University of Melbourne, Parkville, VIC 3010, Australia. Patrick F. James is head of product development, Michael Whitmore was head of process development, Anabel Silva is senior research scientist, Karmen Kong is research assistant, Melanie Schoppet is senior research scientist, Chantelle Blyth is research scientist, Mun Joo Chuei is research scientist, Karen Holden was EM pipeline products manager, and Ian Dixon is managing director, all at Exopharm Ltd. Gregor Lichtfuss is business services manager at Exopharm Ltd. and Honorary Fellow at the Department of Biochemistry and Pharmacology at the University of Melbourne.