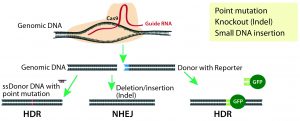
Figure 1: CRISPR/Cas9 system for genome modification in eukaryotic cells; guide RNAs are synthesized specific to the target location to be modified, and they direct the Cas9 nuclease to make double-stranded breaks. A nonhomologous end-joining (NHEJ) repair mechanism ligates the cut ends of the DNA nonspecifically, whereas a homology-directed repair (HDR) mechanism repairs the double-stranded breaks using a donor template with homologous sequences to the cut site.
Transgenic mouse models have been an essential part of biomedical research for many decades. They have provided valuable insights in developmental biology, gene regulation, and our understanding of the genetic basis of human disease. And they play a critical role in drug discovery and development. Traditional methods to generate these mouse models entailed a milieu of disadvantages: e.g., low efficiency, high incidence of undesirable recombination outcomes, randomly and multiply inserted genes of interest, ectopic expression, gene silencing, and insertional mutations (1). The process is labor intensive, requires large colonies of animals, and involves unpredictable deliverables and/or long turnaround times.
Development of targeted gene-editing technologies has greatly improved site-directed mutagenesis and led to generation of better mouse models (knock-in, knock-out, gene tagging, and conditional expression models) with increased efficiency and precision as well as shorter turnaround. Here we provide a brief insight into how two complementary gene editing technologies enable generation of disease-relevant mouse models: clustered regularly interspaced short palindromic repeats (CRISPR) and the TARGATT site-specific DNA integration system.
Advances in Gene-Editing Technologies
Site-Specific Nucleases: The new millennium witnessed development of site-specific gene modification techniques such as zinc finger nucleases (ZFNs), transcription activator-like effector nucleases (TALENs), and the revolutionary CRISPR/Cas9 technology (2). All three technologies use endonucleases that introduce double-stranded DNA breaks (DSBs) at specific sites in a genome. Those breaks are repaired by one of two mechanisms: the more active nonhomologous end-joining repair (NHEJ) or the less active homologous-directed repair (HDR) (Figure 1).
NHEJ-based DNA repair is error prone and results in small nucleotide insertions and deletions (indels) that lead to subsequent frame shifts or deletion mutations and potentially to the loss of gene function. This repair pathway is used for engineering gene-knockout or loss-of-function models. By contrast, HDR-mediated repair is more accurate because it integrates a donor DNA with homology arms to the sequence at the DSB. Therefore, it is used for site-specific gene knock-in and point-mutation models (3).
Development of the CRISPR/Cas9-nuclease system for genome editing in eukaryotic cells (Figure 1) started a new generation of advanced and precise cellular and animal models. This technique uses simple site-specific guide RNAs (gRNAs) as probes to target Cas9 nuclease cleavage. The method has gained popularity because of its simplicity regarding design and delivery of RNA probes, its high specificity and efficiency, its ease of implementation, its relatively low cost, and its rapid turnaround time. But CRISPR outcomes are tainted by off-target mutagenesis that result from NHEJ-mediated indels in nontargeted sites after indiscriminate Cas9 cleavage. CRISPR also has low efficiency for site-specific insertion of large gene fragments for knock-in mouse model generation. However, the technology is still in its infancy, and much effort is being expended to improve its precision and gene-insertion capabilities.
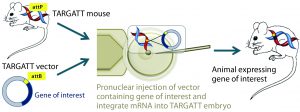
Figure 2A: The TARGATT integrase technology for large-fragment gene knock-in, with an example Cre transgene; method of generating transgenic mouse using TARGATT technology. The TARGATT mouse has been engineered with attP docking sites in a preselected locus of the mouse genome. A donor vector containing a gene of interest and attB sites is coinjected with integrase mRNA into the pronuclei of mouse zygotes. Then the injected zygotes are implanted into pseudopregnant mice to carry to term.
Integrase-Based Method for Gene Knock-In Models: To compensate for the low efficiency of inserting large DNA fragments using CRISPR, researchers developed another avenue of site-specific gene editing technology. The bacteriophage ΦC31 (PhiC31) integrase system enables site-specific integration with higher efficiency because it catalyzes an irreversible reaction between two appropriate attP and attB sites (Figure 2) (4, 5).
In mice, the ΦC31 integrase catalyzes insertion of a gene of interest into a preengineered attP “docking†site at a specific locus (Rosa26 or H11) in the mouse genome (4, 6). The preselected loci are at transcriptionally active and well-characterized chromosomal regions, guaranteeing transgene expression without causing positional effects or insertional mutations.
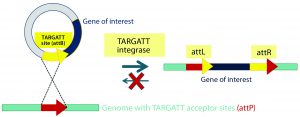
Figure 2B: Integrase catalyzes an irreversible recombination between the attP and attB sites, resulting in two new hybrid sites — attL and attR — that are no longer recognized by the integrase enzyme. Gene integration is stable as a result, and the process is highly efficient in generating transgenic mice.
We adapted the ΦC31-integrase system for our proprietary TARGATT technology and designed it to support insertion of large-fragment DNA (up to 22 kb) with site-specificity and high efficiency. This provides a platform for
- gene over-expression
- reporter gene expression
- humanized animal models
- advanced models with inducible/conditional gene expression in specific tissue and/or at a specific time in combination with the complementary CRISPR technology.
Conditional/Inducible Knockout Models: Mouse models have been an integral part of research and discovery in biomedicine for more than three decades. Presently, humanized mouse models (7), conditional/inducible knockout, and knock-in models are gaining popularity with increasing demand for accurate and relevant models of human genetics and diseases.
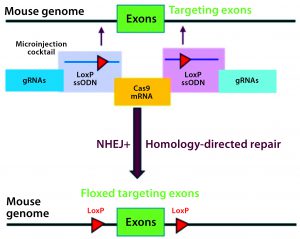
Figure 3A: Conditional knockout strategy in mouse models; two separate CRISPR systems were designed to insert the flanking LoxP sequences at the desired 5′ and 3′ locations of the targeting exons.
Conditional knockout (CKO) animal models circumvent the hurdles of constitutive knockout models such as embryonic lethality, compensatory mechanisms, and undesired phenotypes, and they can mimic human diseases better than the alternative (8). The most commonly used CKO method is the Cre-LoxP system, in which a gene of interest (targeted exons) is flanked by two 34-bp LoxP sequences (also called floxed alleles) at specific genomic sites using CRISPR technology. The LoxP sites serve as a target for the Cre-recombinase, which catalyzes deletion of the floxed exon(s) (Figure 3A).
In one of many CKO mouse models we have generated, we inserted one LoxP at designated locations in each 5′ and 3′ untranslated region (UTR) of a confidential gene of interest. The model generation involved
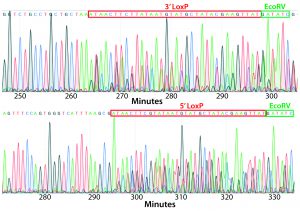
Figure 4: PCR and sequencing results of mice born after embryonic microinjection with CRISPR cocktail; (top) two out of 12 mice were identified as founders and showed the expected fragment shifts for both 5′ and 3′ LoxP insertions. A LoxP insertion at the 5′ site (intron 1) produced a 513-bp PCR fragment (blue box, WT 473 bp), and LoxP insertion at the 3′-targeting site produced a 539-bp PCR fragment (red box, WT 499 bp). The representative chromatogram (bottom) comes from sequence analyses of founder mice confirming LoxP insertion at 5′ and 3′ locations of the desired genomic locus.
microinjecting a mixture of active gRNAs, donor oligonucleotides (ssODNs) as LoxP sequence donors, and qualified Cas9-protein into C57BL/6 embryos. The genotypes of founder mice were screened and confirmed using polymerase chain reaction (PCR) and sequencing of genomic DNA isolated from tail snips. Two out of the 12 mice born were identified as founders for both 5′ and 3′ LoxP insertions (Figure 4).
Technology to Generate Cre-Expressing Mice: Once the “floxed†CKO mice are generated, they can be crossbred with transgenic Cre mice with recombinase expression under the control of a promoter of choice to direct either tissue-specific or ubiquitous expression (Figure 3B).
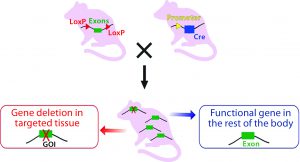
Figure 3B: Crossbreeding the floxed mouse with a Cre-recombinase expressing mouse produces a condition knockout model. The Cre expression is driven by a promoter of choice (yellow triangle, a tissue-specific or ubiquitous promoter). Expressed Cre recombinase deletes the floxed exon(s) spatial-specifically, thereby causing a frame shift in the downstream sequence.
Although conditional knockout mice are easily generated, only a limited number of Cre mice are currently available on the market (8). Other tissue-specific/inducible Cre transgenic mice required to establish a Cre-LoxP breeding pair must be custom engineered. Our TARGATT integrase technology can be used to generate such mice because it supports large DNA fragments containing both a Cre gene and its regulatory elements (promoters, termination sequence, and so on). Knock-in fragments can be inserted into the “attP docking†site in commercially available TARGATT mice (Charles River Laboratories) at a transcriptionally active, safe-harbor locus (Rosa26 or H11), with very high efficiency and guaranteed expression.
In an example project, first we constructed a TARGATT plasmid with a donor fragment containing
- a blood-cell specific promoter
- an improved Cre gene (iCRe)
- a multicistronic element to transcribe a reporter gene
- reporter gene
- a transcription terminator signal.
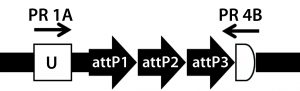
Figure 5A: Transgene integration and genotyping scheme of a TARGATT-Cre transgenic mouse model — genomic sequence with attP docking site at H11 locus
A cocktail of the donor plasmid and in vitro transcribed ΦC31 integrase mRNA was microinjected into the pronuclei of C57BL/6 embryos that had attP sites engineered into the H11 safe-harbor locus. We then transferred the resulting embryos into pseudopregnant surrogate mice. Out of three mice later born, we used PCR-based genotyping (Figure 5) to identify two as founders with the Cretransgene insertion.
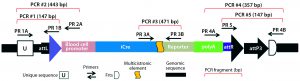
Figure 5B: Transgene integration and genotyping scheme of a TARGATT-Cre transgenic mouse model — founder genotype with transgene
When such a Cre-mouse is mated with a mouse carrying a “floxed†gene of interest, the Cre expression (controlled by promoter of choice) results in targeted deletion of the floxed allele. Cre expression has minimal unwanted effects in these animals because the mouse genome contains no endogenous LoxP sites, which provides an ideal background for site-specific recombination.
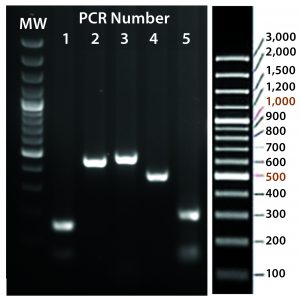
Figure 5C: Transgene integration and genotyping scheme of a TARGATT-Cre transgenic mouse model — identification of founders with transgene insertion by PCR using primer pairs (lanes 1–5). PCR #1 recognizes site-specific insertion at 5′ insertion site (attL formation), PCR #2 detects insertion of transgene promoter at 3′ site, PCR #3 recognizes iCre and reporter genes, PCR #4 detects transgene insertion at 3′ site of H11 locus, and PCR #5 identifies the attR sequence formed after ΦC31 mediated insertion. (GeneRuler 100-bp Plus DNA marker is used in lane MW.)
Summary
Genetically engineered cellular and animal models are essential preclinical research tools in academic and biotechnology laboratories. Demand is increasing for advanced models with improved specificity. Until recently, gene-editing technology has struggled to keep pace to support researchers’ requirements. But several technologies have risen in the past decade to revolutionize genomic research and preclinical studies — and they may even offer potential for gene therapy in humans. They have helped scientists engineer mouse models that mimic human genetics and provide reliable interpretation of human physiology and diseases.
Two such technologies — the nuclease-based CRISPR and integrase-based TARGATT large gene knock-in genome editing technologies — are malleable, site-specific, and efficient. Both offer consistent and reliable gene-editing results. These complementary techniques together broaden the scope of mouse-model generation and can help researchers generate precise disease models in mice, thus advancing biomedical research overall.
About the Company |
Founded in 2008, Applied StemCell (ASC) is based in the San Francisco Bay area. The company focuses on gene-editing and stem-cell innovations for biomedical research and biotechnology. In addition to the proprietary TARGATT gene-editing platform, ASC also has in-licensed the CRISPR gene-editing platform and successfully combined the two in gene-editing services for highly specific transgene expression. ASC’s service platform applies to basic research, drug discovery, bioprocessing, bioproduction, and preclinical testing applications.
The company currently focuses on:
|
References
1 Rocha-Martins M, et al. From Gene Targeting to Genome Editing: Transgenic Animals Applications and Beyond. Anais Acad. Brasil. Ciên. 87(2) 2015: 1323–1348.
2 Ishida K, Gee P, Hotta A. Minimizing Off-Target Mutagenesis Risks Caused By Programmable Nucleases. Int. J. Molec. Sci. 16(10) 2015: 24751–24771; doi:10.3390/ijms161024751.
3 Gupta RM, Musunuru K. Expanding the Genetic Editing Tool Kit: ZFNs, TALENs, and CRISPR-Cas9. J. Clin. Invest. 124(10) 2014: 4154–4161.
4 Tasic B, et al. Site-Specific Integrase-Mediated Transgenesis in Mice Via Pronuclear Injection. Proc. Nat. Acad. Sci. USA 108(19) 2011: 7902–7907; doi:10.1073/pnas.1019507108.
5 Zhu F, et al. (2014). DICE, an Efficient System for Iterative Genomic Editing in Human Pluripotent Stem Cells. Nucl. Acids Res. 42(5) 2014: e34; doi:10.1093/nar/gkt1290.
6 Rossant J, Nutter LMJ, Gertsenstein M. Engineering the Embryo. Proc. Nat. Acad. Sci. USA 108(19) 2011: 7659–7660; doi:10.1073/pnas.1104844108.
7 Ito R, et al. Current Advances in Humanized Mouse Models. Cellul. Molec. Immunol. 9(3) 2012: 208–214; doi:10.1038/cmi.2012.2.
8 Doyle A, et al. The Construction of Transgenic and Gene Knockout/Knockin Mouse Models of Human Disease. Transg. Res. 21(2) 2012: 327–349; doi:10.1007/s11248-011-9537-3.
Corresponding author Neeraja Parameswaran is marketing and business development manager, Lanna Cox is business development development manager, Jinling Li is vice president of operations, Luping Huang is director of business development, and corresponding author Ruby Yanru Chen-Tsai is chief scientific officer, senior vice president, and cofounder of Applied StemCell, Inc., Milpitas, CA 95035; 1-866-497-4180, fax 1-650-800-7179; info@appliedstemcell.com; www.appliedstemcell.com.