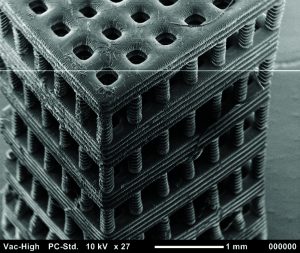
Figure 4: Tissue scaffold fabricated with stereolithography from a biocompatible and biodegradable polymer. Chartrain NA, et al. Microstereolithography of Tissue Scaffolds Using a Biodegradable Photocurable Polyester. International Solid Freeform Fabrication Symposium: Austin, TX, 2015 (in press).
Commonly referred to as three-dimensional (3D) printing, additive manufacturing encompasses a set of technologies that fabricate objects in an additive way, layer by layer, rather than conventional means of fabrications that generally subtract unwanted material from a larger block. Precise control over material placement allows 3D printing to fabricate objects that otherwise would not be manufacturable. Although many of these technologies have been around for two or three decades, recently they have received a significant amount of attention from industry, academia, and the general public. 3D printed components are now fabricated as end-user parts in the automotive, biomedical, and aerospace industries. However, the potential use of 3D printing to engineer tissues might be even more exciting.
Tissue engineering repairs or replaces damaged or diseased tissue though a combination of cells, scaffolds, and growth or differentiation cues (1, 2). Cells can be from allogeneic sources, although autologous cells are preferred to eliminate the possibility of adverse immune responses (2). Both pluripotent stem cells and differentiated cells have been seeded onto scaffolds. Tissue scaffolds are 3D cellular devices with large surface areas that facilitate cell attachment and growth. Scaffolds must provide mechanical support to cells while allowing nutrients and waste to diffuse throughout (3).
Perhaps the greatest challenge in tissue engineering over the past two to three decades has been incorporating vasculature to provide more effective movement of nutrients and waste through a thick scaffold (4). Planar or tubular tissues such as skin and blood vessels do not require an extensive vascular network because nutrients can diffuse through thin tissue. However, overcoming the vascularization challenge is crucial for regeneration of solid organs (e.g., liver, kidney, and heart) in which diffusion limitations cause cell death at the scaffold center (4).
Tissue scaffolds also can include a number of reagents that can encourage cell differentiation and/or spatial organization. Growth factors often are used: e.g., bone morphogenetic protein (BMP-2), which encourages osteoblastic differentiation (5). Some researchers also have been exploring the use of mechanical and electrical stimulation to encourage development of skeletal and cardiac muscle tissue (6).
In the 1990s, fabrication of tissue scaffolds for regenerative medicine began in earnest with the development and improvement of several polymer processing techniques that allowed fabrication of porous structures from biocompatible and biodegradable materials (7, 8). Techniques such as gas foaming, particulate leaching, and electrospinning are still the most popular methods for fabricating tissue scaffolds, in part because of the flexibility they offer in material selection (7, 9). Notably, some researchers have replaced planar and tubular tissues in both animal and human subjects (10–12). However, because these processing techniques create a stochastic arrangement of pores in the scaffolds, the fabricated constructs have no hierarchical structure that would allow formation of a vascular network. Thus, regeneration of solid tissues has remained elusive (2).
Additive manufacturing can control precise material placement in three dimensions and is well suited to fabrication of hierarchical scaffolds for tissue engineering. However, 3D printing has its own material and resolution limitations that so far have hindered its widespread adoption. Below, we review the technologies most often used in additive fabrication of tissue scaffolds and provide a brief outlook of the opportunities and challenges currently facing the field.
3D Printing Technologies for Tissue Engineering
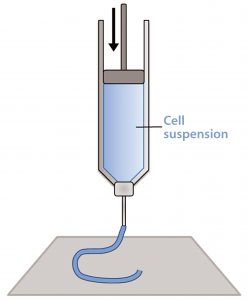
Figure 1: Schematic of a piston extrusion bioprinter; a cell suspension, molten thermoplastic, or viscous liquid is loaded into a syringe and extruded.
Additive manufacturing technologies can fabricate objects from a range of polymers, metals, ceramics, and composite materials. However, tissue scaffolds and other biologics are fabricated from polymers using three primary techniques: material extrusion, inkjet bioprinting, and stereolithography (13).
Material extrusion, sometimes referred to as fused deposition modeling (FDM) or simply extrusion, pushes a bead of viscous liquid or molten material through a thin nozzle to lay down tracks of material one atop another (14). This most often comes through melting and extruding a thermoplastic filament (e.g., polylactic acid), but thermosets, cell suspensions, and UV curing materials can be loaded into syringes and extruded as well (Figure 1). In fact, most extrusion systems contain more than one “print head†so that multiple materials and even several types of cells can be placed into a single object (15).
One print head can be used to deposit a hydrogel or biodegradable thermoplastic such as polycaprolactone (PCL), for example, while a second print head deposits a cell suspension. The scaffold provides mechanical support for the cell suspension, which otherwise would not be able to hold its shape during printing. Over an extended period, the scaffold dissolves and is replaced by extracellular matrix.
With piston, pneumatic, and screw extruders, extrusion-based bioprinters can fabricate parts using materials with an extremely wide range of viscosities (2). Extrusion-based bioprinters also can fabricate parts with higher cell densities than other 3D printing techniques can provide, which is essential for tissue formation and physiological function.
Although extrusion can fabricate using cells and other materials at the same time, printing cell-sized features can be a challenge. Extruding a material through a small needle requires either high pressure or a long time. Shear stresses can damage cells and even rupture their membranes (2, 16). Cells that do survive the printing process should not be so extensively damaged that they are unable to perform their biological functions.
Larger nozzle diameters or lower pressures can be used at the expense of longer print times and lower resolutions. Extruded material also must gel relatively quickly so that the structure does not collapse (17). However, gelling too fast will clog the nozzle. Many materials printed to provide cell support are either thermoplastics that require high processing temperatures that can damage cells or hydrogels that cannot provide structural integrity to scaffolds more than a few millimeters tall. Finally, cells must be kept viable in both the dispensing syringe and the partially printed scaffold throughout the printing process and incubation.
Despite those challenges, material extrusion has been used successfully to engineer a number of tissues and devices. For example, researchers at the University of Michigan fabricated degradable tracheal splints for infants with severe tracheobronchomalacia in which collapse of the trachea is acutely life threatening (18). Made of biodegradable polycaprolactone (PCL) polymer, the splints are designed to grow with a child as his or her trachea increases in diameter. Another group at Cornell has used extrusion bioprinting to fabricate an aortic valve conduit that incorporates two distinct cell types (15). Anthony Atala at the Wake Forest Institute for Regenerative Medicine demonstrated a bioprinted kidney during a TED Talk; however, his institute was quick to point out that it was only a preliminary, nonfunctional model (2). Incorporation of vasculature and function in a whole kidney still requires significant research before becoming a reality.
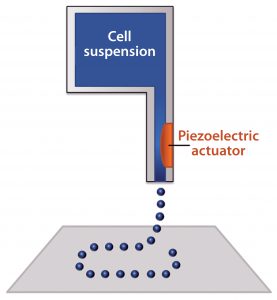
Figure 2: Schematic of an inkjet bioprinter using a piezoelectric component; a voltage is applied to create a pressure gradient near the nozzle, which ejects small droplets of material
Inkjet bioprinting uses the same type of print heads found in 2D inkjet printers mounted on a three-axis gantry (19). The print heads have ink reservoirs and a very small nozzle through which small droplets are ejected using thermal excitation or a pressure pulse delivered by a piezoelectric component (Figure 2). Thermal print heads work by applying a quick heat pulse to a chamber closest to the nozzle, creating a vapor bubble. Pressure from that vapor bubble ejects a small droplet of ink. Piezoelectric print heads instead directly apply pressure to the fluid. When a voltage is applied to the piezoelectric component, it changes shape, and the resulting pressure ejects the ink droplet (20).
In both types of inkjet printing, UV curable materials and those that cure over time through gelation or solvent evaporation can be used (17). Researchers often purchase commercially available print heads, then remove the ink and replace it with cells and material (“bioinkâ€). Thus, inkjet bioprinting is a popular technique among academics because of its low cost, high print speeds, and good resolution. Multiple inkjet heads mounted on a three-axis gantry can deposit materials and different cell types to create very complex tissue scaffolds. Intricate patterns can be formed from droplets that can contain just a few cells each. Although inkjet printed cell densities are not as high as those from extrusion bioprinting, more cells can survive this gentler printing process (2, 16, 17).
Although extrusion can print materials with a broad range of rheological properties, inkjet bioprinting can print only materials with relatively low viscosities because of its very small nozzle sizes. However, as with extrusion bioprinting, shear stresses during jetting can have deleterious effects on cells. In addition, low-viscosity bioinks must gel or polymerize quickly to maintain printed resolution and provide structural integrity for the scaffold. Many researchers are now synthesizing new bioinks based on both natural and synthetic polymers with jettable viscosities that provide structural integrity (17).
Inkjet printing has been used to print a number of bioinks containing many different cell types. Boland et al. fabricated a branched, chambered structure reminiscent of cardiac tissue made from an alginate-based bioink (21). That group also demonstrated simultaneous printing of endothelial cells with fibrin for creation of microvascularized constructs (22). Others also have successfully combined mesenchymal stem cells with a UV-crosslinkable hydrogel material to print tissue constructs for bone and cartilage regeneration (23).
Stereolithography (vat photopolymerization) is a 3D printing technique that uses patterned light to polymerize a liquid photopolymer selectively, layer by layer (Figure 3) (24). Photopolymers are materials that crosslink or cure to form a solid when exposed to visible or UV light. They are widely used in the microelectronics industry and as dental fillings. In stereolithography, such photopolymers can be solidified either by rastering a laser over the liquid-resin surface or by projecting an entire image at once (mask-projection stereolithography) (25). The resulting very thin layer adheres to a build stage placed just below the resin surface. The build stage then moves downward by one layer thickness, submerging the previously cured layer by a thin layer of liquid resin. That process is repeated layer by layer until an entire part has been fabricated. Companies including Formlabs and Carbon3D have commercialized a variation of this technique in which light instead irradiates a resin vat through a window from below.
Because of its scalability and excellent resolution, stereolithography is used to fabricate nearly all in-the-ear hearing aids and millions of Invisalign teeth aligners. However, no commercially available stereolithography materials are both biocompatible and biodegradable. To fabricate degradable tissue scaffolds, researchers must synthesize their own printable materials (24). Several materials have been reported, but none have been subject to extensive in vivo tests for multiple tissue types. Additional concerns remain over toxicity of the photoinitiators and uncured resin. Finally, cells must be seeded onto a scaffold after printing rather than during the printing process.
Despite those challenges, stereolithography has been used to fabricate tissue scaffolds with feature sizes on the micron scale (26). In addition, design and synthesis of novel stereolithography resins for building tissue scaffolds is the subject of ongoing research at several institutions.
The resolution and geometric flexibility afforded by this technique allows very complex structures to be fabricated (Figure 4). It could enable researchers to study the effects that pore shape and scaffold geometry have on cell response (27). Even more important, the fabrication of tubular constructs within a scaffold might mimic vascularity that could improve nutrient flow throughout a thick tissue scaffold. Researchers have used stereolithography to fabricate tissue scaffolds with excellent resolution from a number of biocompatible polymers. Some have been seeded with endothelial cells, osteoblasts, chondrocytes, and breast cancer cells (24, 26, 28–30).
Future OutlookÂ
Although the advent of widespread organ printing is not as close as some media hype might suggest, important advances have been made in using 3D printing to engineer tissues. Over the past two decades, multiple 3D printing techniques have demonstrated their utility in fabricating small tissue scaffolds or biological constructs. However, significant engineering challenges have yet to be overcome. Creating new materials is a lengthy process, as is modifying existing ones to make them compatible with the printing processes. In addition, printers cannot yet pattern material and cells at scales compatible with a human body. The overall construct dimensions are rarely over a centimeter across, and the heterogeneous patterning of cells and materials below 50 µm remains challenging.
Much work in the next few years will continue to focus on materials and resolution. However, eventually the research focus must shift to other challenges such as quality control and scaffold design. The US Food and Drug Administration (FDA) has issued guidance for 3D printing of medical devices such as surgical guides and orthopedic implants but has not yet provided guidance for 3D printed biologics that include cells and tissue. Not only will regulations be needed, but the biopharmaceutical industry will have to determine how to ensure that a 3D-printed tissue construct will remain viable after implantation and perform as expected.
Currently, researchers use biomimetic design, and inspiration to fabricate scaffolds and tissue constructs. However, it is difficult to say whether those are the most optimal structures onto which new cells can be cultured. It is possible that alternative architectures would be more favorable to the growth and differentiation of cells. Tissues may then reorganize themselves into an architecture more akin to that found in our bodies.
Finally, incorporating vascularity into engineered tissues is of crucial importance. Without including a network that allows for the flow of blood and nutrients into and waste out of engineered tissue, developers will be unable to fabricate solid tissues and organs.
Additive manufacturing has tremendous potential to create engineered tissues that could improve life for thousands of patients waiting for transplants. Engineered tissues made with 3D printing even could be used to address medical conditions that are not presently treated using transplants: e.g., diabetic ulcers, tissue excision related to cancer, and joint replacement. Although traditional tissue fabrication technologies may be adequate for creating planar and tubular tissues, 3D printing may be the only viable method for creating solid organs such as kidneys, livers, and hearts.
References
1 Atala A. Engineering Organs. Curr. Opin. Biotechnol. 20(5) 2009: 575–592.
2 Murphy SV, Atala A. 3D Bioprinting of Tissues and Organs. Nat. Biotechnol. 32(8) 2014: 773–785.
3 Hollister SJ, Maddox RD, Taboas JM. Optimal Design and Fabrication of Scaffolds to Mimic Tissue Properties and Satisfy Biological Constraints. Biomaterials 23(20) 2002: 4095–4103.
4 Novosel EC, Kleinhans C, Kluger PJ. Vascularization Is the Key Challenge in Tissue Engineering. Adv. Drug Delivery Rev. 63(4–5) 2011: 300–311.
5 Woo J, et al. Bone Regeneration Using a Microstereolithography-Produced Customized Poly (Propylene Fumarate)/Diethyl Fumarate Photopolymer 3D Scaffold Incorporating BMP-2 Loaded PLGA Microspheres. Biomaterials 32(3) 2011: 744–752.
6 Tandon N, et al. Electrical Stimulation Systems for Cardiac Tissue Engineering. Nat. Protoc. 4(2) 2009: 155–173.
7 Langer R, Vacanti J. Tissue Engineering. Science 260(5110) 1993: 920–926.
8 Hutmacher DW. Scaffolds in Tissue Engineering Bone and Cartilage. Biomaterials 21(24) 2000: 2529–2543.
9 Mikos AG, et al. Preparation and Characterization of Poly(l-Lactic Acid) Foams. Polymer 35(5) 1994: 1068–1077.
10 De Filippo RE, Yoo JJ, Atala A. Urethral Replacement Using Cell Seeded Tubularized Collagen Matrices. J. Urol. 168(4, Pt 2) 2002: 1789–1793.
11 Raya-Rivera AM, et al. Tissue-Engineered Autologous Vaginal Organs in Patients: A Pilot Cohort Study. Lancet 384(9940) 2014: 329–336.
12 Catalano E, et al. Tissue-Engineered Skin Substitutes: An Overview. J. Artif. Organs.16(4) 2013: 397–403.
13 Melchels FPW, et al. Additive Manufacturing of Tissues and Organs. Progr. Polymer Sci. 37(8) 2012: 1079–1104.
14 Gibson I, Rosen D, Stucker B. Additive Manufacturing Technologies: Rapid Prototyping to Direct Digital Manufacturing. Springer: New York, NY, 2010.
15 Duan B, et al. 3D Bioprinting of Heterogeneous Aortic Valve Conduits with Alginate/Gelatin Hydrogels. J. Biomed. Mater. Res. A 101(5) 2013: 1255–1264.
16 Seol YJ, et al. Bioprinting Technology and Its Applications. Eur. J. Cardiothorac Surg. 46(3) 2014: 342–348.
17 Skardal A. Bioprinting Essentials of Cell and Protein Viability. Essentials of 3D Biofabrication and Translation. Atala A, Yoo JJ, Eds. Academic Press: Cambridge, MA, January 2015: 1–17.
18 Morrison RJ, et al. Mitigation of Tracheobronchomalacia with 3D-Printed Personalized Medical Devices in Pediatric Patients. Sci. Transl. Med. 7(285) 2015: 285ra64.
19 Mironov V, et al. Organ Printing: Computer-Aided Jet-Based 3D Tissue Engineering. Trends Biotechnol. 21(4) 2003: 157–161.
20 Xu T, et al. Principles of Bioprinting Technology. ResearchGate December 2014: 67–79.
21 Xu T, et al. Fabrication and Characterization of Bio-Engineered Cardiac Pseudo Tissues. Biofabrication 1(3) 2009: 35001.
22 Cui X, Boland T. Human Microvasculature Fabrication Using Thermal Inkjet Printing Technology. Biomaterials 30(31) 2009: 6221–6227.
23 Gao G, et al. Improved Properties of Bone and Cartilage Tissue from 3D InkjetBioprinted Human Mesenchymal Stem Cells By Simultaneous Deposition and Photocrosslinking in PEG-GelMA. Biotechnol. Lett. 37(11) 2015: 2349–2355.
24 Lambert P, et al. Mask Projection Microstereolithography of Novel Biocompatible Polymers. International Solid Freeform Fabrication Symposium: Austin, TX, 2014. University of Texas: Austin, TX; 974–990.
25 Lambert PM, Williams CB. Design Considerations for Mask Projection Microstereolithography Systems. Solid Freeform Fabrication Symposium: 8–10 August, Austin, TX.
26 Sirrine JM, et al. 3D-Printable Biodegradable Polyester Tissue Scaffolds for Cell Adhesion. Australian J. Chem. 68(9) 2015: 1409.
27 Melchels FP, et al. Effects of the Architecture of Tissue Engineering Scaffolds on Cell Seeding and Culturing. Acta Biomater. 6(11) 2010: 4208–4217.
28 Melchels FPW, Feijen J, Grijpma DW. A Review on Stereolithography and Its Applications in Biomedical Engineering. Biomaterials 31(24) 2010: 6121–6130.
29 Melchels FP, et al. The Influence of the Scaffold Design on the Distribution of Adhering Cells After Perfusion Cell Seeding. Biomaterials 32(11) 2011: 2878–2884.
30 Gauvin R, et al. Microfabrication of Complex Porous Tissue Engineering Scaffolds Using 3D Projection Stereolithography. Biomaterials 33(15) 2012: 3824–3834.
Corresponding author Nicholas A. Chartrain (nickchar@vt.ed) is a PhD student in the Macromolecules Innovation Institute and the department of materials science and engineering; Christopher B. Williams (cbwilliams@vt.edu) is associate director of the Macromolecules Innovation Institute and a professor in the departments of materials science and engineering and mechanical engineering; Abby R. Whittington (awhit@vt.edu) is a professor in the Macromolecules Innovation Institute and the departments of materials science and engineering and chemical engineering; all at Virginia Tech, 1075 Life Sciences Circle, Suite 110, Blacksburg, VA 24060; 1-540-231-6824; www.mii.vt.edu.