In Part 1 of this report, we described our development of a high-throughput assay for analyzing monoclonal antibody (MAb) glycans and how we used it to evaluate the effects of medium supplements on galactosylation of MAbs produced by two different cell lines (1). This month, we examine galactosylation of a MAb produced by a third cell line. A discussion follows on the benefits of this high-throughput assay before we highlight the similarities and differences in galactosylation among the three MAbs in our study.
Results for Cell Line 3
Our study on the third cell line was similar to the studies for cell lines 1 and 2. Cell line 3 was cultivated in shake flasks with Gibco Dynamis medium and supplemented with EfficientFeed C+ supplement (EFC+), both from Thermo Fisher Scientific. Maximum cell densities ranged from 1.7 to 2.5 × 107 cells/mL, and culture viabilities at harvest were 42–78%. Despite those wide ranges, the galactose-enhancing supplements had little effect on MAb production in this case: The control culture contained 480 mg/mL, whereas the treated cultures produced 490–550 mg/mL.
We supplemented these cultures with feeds designed to enhance galactosylation: EX-CELL Glycosylation Adjust (Gal+) supplement from MilliporeSigma; Gibco Glycan Tune C+ (Thermo Fisher Scientific) starting on day 3 or day 8 of culture; or one of four concentrations of a mixture containing uridine, manganese (Mn), and galactose. The first three mixtures were equivalent to the low, middle, and high doses of uridine–Mn–galactose used to treat cell line 1; the fourth dose used here is identified as the “higher dose” mixture in Table 1.
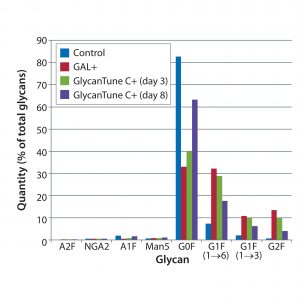
Figure 2: Effects of commercial supplements on glycosylation of MAb 3 at culture harvest; control is that used in Figure 1.
G0F content of MAb 3 at harvest was about 80%, greater than its content in MAbs 1 and 3 (which were about 60% and 70%, respectively). Despite the greater G0F content of MAb 3, the uridine–Mn–galactose mixtures and commercial supplements enhanced MAb3 galactosylation (Figures 1 and 2). However, by contrast with MAbs 1 and 2, all supplements enhanced galactosylation — not just of G1F(1→6), but also G1F(1→3) and G2F. The same control is shown in both figures.
The time course for MAb galactosylation depended on the cell line and culture supplement. Examination of the data in Figures 3 and 4 confirms that the relative amount of G0F glycan is 80% at harvest because data from the preceding days lead to 80%. Supplements reduced the quantity of G0F glycan to <40% of the total glycan and increased the quantity of G2F glycan from ~1% to amounts ranging from 4% to 14% of the total glycan. It appears that the extent of galactosylation can be controlled by adding GlycanTune C+ supplement to cultures starting on day 3 or day 8. One observation in common with the previously described studies was a larger change in G1F(1→6) galactosylation than in G1F(1→3) galactosylation. The controls in Figures 3 and 4 are from Figures 1 and 2, respectively.
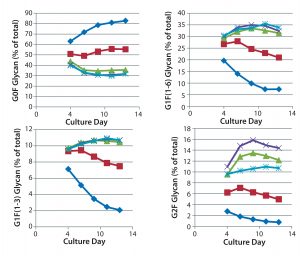
Figure 3: Effect of uridine–Mn–galactose mixtures on galactosylation of MAb 3 glycans during cultivation; ♦–♦ Efficient Feed C+ (EFC+ = control); â– –â– EFC+ with uridine–Mn–galactose (low dose); â–˛–â–˛ EFC+ with uridine–Mn–galactose (mid-dose); Ă—–Ă— EFC+ with uridine–Mn–galactose (high dose); *–* EFC+ with uridine–Mn–galactose (higher dose); day 13 data are from Figure 1.
Discussion
High-Throughput (HTP) Assay: The biopharmaceutical industry needs HTP assays to quantify glycan moieties on proteins made by recombinant means, including MAbs (2). Our assay meets the requirements for rapidity, precision, and the capacity to test a large number of samples at a time. The assay uses a suspension of negatively charged magnetic beads to adsorb glycans released from MAb glycoproteins by peptide-N-glycosidase F (PNGase F). Glycans adsorbed to beads are separated from the fluid phase without the need for pipetting. Glycan isolation and labeling can be accomplished in a day; samples can be analyzed overnight using capillary electrophoresis.
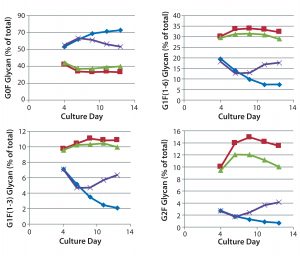
Figure 4: Effect of commercially obtained supplements designed to enhance glycosylation on the galactosylation of MAb 3 glycans during cultivation; ♦–♦ Efficient Feed C+ (EFC+ = control); â– –â– EFC+ with GAL (low dose); â–˛–â–˛ EFC+ with GlycanTune C+ (day 3); Ă—–Ă— EFC+ with GlycanTune C+ (day 8); day 13 data come from Figure 2.
Glycan analysis based on fluorescent labeling has been in widespread use since the 1990s. Although the methods of analysis have remained constant, the methods of preparing glycans for analysis have varied considerably. Glycoproteins have been denatured before treatment with PNGaseF (3–5). Enzyme treatments typically were performed at 37 °C for 16–24 hours (6, 7). Methods used to isolate glycans from protein have been lengthy in some cases (3, 4, 8). Reaction of glycans with fluorescent molecules such as 8-aminopyrene-1,3,6-trisulfonic acid (APTS) under acidic reducing conditions often were performed overnight (4, 9). Reaction mixture clean-up ranged from dilution with water (10) to column chromatography and concentration of samples (5, 7). Teams led by Royle and Ruhaak used 96-well plates (10, 11). A method developed by Varadi et al. shortened and simplified the method for preparation of MAb samples for glycan analysis by shortening incubation times and using charged magnetic beads to effect separations (12).
Modulation of MAb Galactosylation: Changes to the cultivation medium and cell line affected MAb galactosylation. The extent of GlcNAc, Gal, and NANA addition depends on the concentration of nucleotide sugars in Golgi vesicles and on activities of mannosidases and glycosyltransferases (13–15). The use of two commercial medium supplements designed to enhance glycosylation — with a mixture of uridine, MnCl2, and galactose — increased MAb galactosylation. Uridine and galactose are precursors of uridine diphosphogalactose (UDP-galactose), which donates galactose to glycans, and Mn is a cofactor for an enzyme that synthesizes it. Increased galactosylation of MAbs in our studies reported herein was similar to that seen in other studies that used similar mixtures of uridine, MnCl2, and galactose.
Gramer et al. supplemented cultures with increasing concentrations of uridine-MnCl2-galactose at a molar ratio of 1:0.002:5 (16). MAb galactosylation increased from 3% to 21% when the supplement concentration increased from 0 to 8Ă—, which was nearly equivalent to the control and low-dose mixture we used. Further increases in concentration from 8Ă— to 20Ă— resulted in a minimal increase in galactosylation from only 21% to 23%; those findings were consistent with ours obtained using mid- and high-dose mixtures. With a second cell line, galactosylation increased from 0% to 29% when supplement concentration was increased from 0 to mid-dose (17). And Fan et al. showed that galactosylation was limited by UDP-galactose synthesis, which in turn was linked to low concentrations of glucose and glutamine in culture media (18). Addition of 20 mM galactose to the medium increased the galactose content of IgG glycans from 14% to 25% (19).
Adding manganese and galactose increased the galactose content of MAb glycans, suggesting that the two compounds are rate limiting. Concentrations of manganese and galactose are increased enough to bring them near their Km values for UDP-galactosyltransferase (20). Km and optimal concentrations for UDP-galactose are in the millimolar range. Thus, the supplement mixture brings both compounds into a relevant range for galactosyltransferase.
Preferential Galactosylation on the Glycan’s α1,6 Arm: Our results showed preferential galactosylation of the glycan’s α1,6 arm over its α1,3 arm. Because the glycans face inward, galactosylation is limited by inaccessibility of GlcNAc on the molecule to the galactosyltransferase enzyme. In addition, galactosylation and sialylation increased when two phenylalanines at positions 241 and 243 were changed because the phenylalanines contributed to stabilizing glycan–protein interactions through hydrophobic CH–π interactions (21, 22). The effect of steric interference is not limited to IgG; it has been shown to affect the glycosylation of Sindbis virus glycoproteins (23), carboxypeptidase Y, and invertase (24).
Some data are consistent with preferential galactosylation of the α1,6 arm. When both arms were galactosylated, nuclear magnetic resonance (NMR) scanning of the solution showed that both arms were mobile at physiological temperature (23, 25, 26). Movement of the galactosylated α1,6 arm was more restricted than that of the α1,3 arm, indicating a greater interaction between the α1,6 arm and the protein, which is in part attributable to galactose–protein interaction (26, 27). However, removing the galactose increased the mobility of the α1,6 arm and altered the glycan’s interaction with the protein. It also increased hydrogen bonding between the α1,3 arm and the protein, perhaps making the arm less accessible to galactosyltransferase or UDP-galactose.
Fortunato and Colina found that the α1,6 arm had greater mobility than was originally thought (26). They also used X-ray crystallographic structures and molecular simulations to examine the motions of galactosylated and nongalactosylated glycans on IgG molecules. When nongalactosylated, the α1,6 arm showed more mobility than the α1,3 arm. That resulted from interactions between the terminal GlcNAc and the protein. There was little interaction of the terminal GlcNAc on the α1,6 arm with the polypeptide.
The contemporary studies cited above are consistent with earlier in vivo and in vitro studies — in particular, indicating favored galactosylation of the α1,6 arm and suppressed galactosylation of the α1,3 arm. In a study using nongalactosylated glycopeptides from a human IgG myeloma, NMR data showed that 17% of the α1,6 arms were galactosylated, whereas the α1,3 arms had no galactose (28). Galactosyltransferase from porcine mesenteric lymph node (EC 4.2.1.38) added galactose to the terminal GlcNAc of one arm on glycopeptides — the more mobile α1,6 arm — and slowed incorporation of galactose on the α1,3 arm (29). Thus, there is more G1F(1→6) glycan than G1F(1→3).
Preferential galactosylation of a terminal GlcNAc on a β1,6 arm was observed during studies using the branched trisaccharide GlcNAcβ1→3(GlcNAcβ1→6)Gal (30) Galactosyltransferase from bovine colostrum added galactose to the α1,6 arm 20× faster than to the α1,3 arm. In just one report, galactosylation of the α1,3 arm was favored over the α1,6 arm. Purified rat liver Golgi UDP-galactose:α-glucosamine β-1,4galactosyltransferase incorporated more galactose onto the α1,3 arm of glycopeptides in an in vitro system (31). Because all such studies have used glycopeptides or glycans as galactose acceptors, it is possible that not all glycan–protein interactions seen in more recent studies were present.
Structural and Physiologic Roles of MAb Galactosylation: Galactosylation of the IgG glycan affects the conformation of the CH2 domain by keeping the two polypeptide chains in an “open” state. Galactose, along with other residues in a glycan, interacts with amino acids predominantly through CH–π interactions to stabilize the polypeptide in a conformation that facilitates antibody binding to Fcγ receptors, to the complement component C1q, and to FcRn receptors. Galactosylation thus has a role in modulating the effector functions of IgG, in particular by attenuating inflammation and enhancing complement-dependent cytotoxicity.
Functionally, galactosylation extends the half-life of IgG in circulation by preventing interactions between terminal GlcNAc residues with the mannose receptor (32). Terminal galactose residues can affect complement-dependent cytotoxicity (33). Uridine 5′-triphosphate (UTP) and UDP-galactose synthetases were limiting to Chinese hamster ovary (CHO) cells in fed-batch cultures with serum-free Roswell Park Memorial Institute (RPMI) medium (34). The immune response — in particular, the quelling of inflammation — depends on the galactosylation of IgG (35). Inflammation is modulated by at least two mechanisms: In the first, the association of galactosylated IgG1 with FcγRIIB and dectin-l lowers complement C5a receptor functions (36). In the second case, galactosylated IgG binds to blood dendritic cell antigen 2 on plasmacytoid dendritic cells to suppress inflammation (37).
Acknowledgment
The collaboration of Rachel Rivera, who freely shared her knowledge of glycan analysis and expertise in operating the capillary electrophoresis instrument, was critical to the success of this work.
References
1 Anderson R, et al. Enhanced Galactosylation of Monoclonal Antibodies: Using Medium Supplements and Precursors of UDP-Galactose, Part 1. BioProcess Int. 16(1–2) 2018: 32–39.
2 Read EK, Park JT, Brorson KA. Industry and Regulatory Experience of the Glycosylation of Monoclonal Antibodies. Biotechnol. Appl. Biochem. 58(4) 2011: 213–219.
3 Chen FA, Evangelista RA. Profiling Glycoprotein N-Linked Oligosaccharide By Capillary Electrophoresis. Electrophoresis 19(15) 1998: 2639–2644; doi:10.1002/elps.1150191512.
4 Guttman A, Pritchett T. Capillary Gel Electrophoresis Separation of High-Mannose Type Oligosaccharides Derivatized By 1-Aminopyrene-3,6,8-Trisulfonic Acid. Electrophoresis 16(1) 1995: 1906–1911; doi:10.1002/elps.11501601314.
5 Hamm M, Wang Y, Rustandi RR. Characterization of N-Linked Glycosylation in a Monoclonal Antibody Produced in NS0 Cells Using Capillary Electrophoresis with Laser-Induced Fluorescence Detection. Pharmaceuticals 6(3) 2013: 393–406; doi:10.3390/ph6030393.
6 Raju TS, et al. Species-Specific Variation in Glycosylation of IgG: Evidence for the Species-Specific Sialylation and Branch-Specific Galactosylation and Importance for Engineering Recombinant Glycoprotein Therapeutics. Glycobiol. 10(5) 2000: 477–486; doi:10.1093/glycob/10.5.477.
7 Ruhaak LR, et al. Optimized Workflow for Preparation of APTS-Labeled N-Glycans Allowing High-Throughput Analysis of Human Plasma Glycomes Using 48-Channel Multiplexed CGE-LIF. J. Proteome Res. 9(12) 2010: 6655–6664; doi:10.1021/pr100802f.
8 Szabo Z, et al. Improved Sample Preparation Method for Glycan Analysis of Glycoproteins By CE-LIF and CE-MS. Electrophoresis 31(8) 2010: 1389–1395; doi:10.1002/elps.201000037.
9 Gennaro L, Salas-Solano O. On-Line CE-LIF-MS Technology for the Direct Characterization of N-Linked Glycans from Therapeutic Antibodies. Anal. Chem. 80(10) 2008: 3838–3845; doi:10.1021/ac800152h.
10 Royle L, et al. HPLC-Based Analysis of Serum N-Glycans on a 96-Well Plate Platform with Dedicated Database Software. Anal. Biochem. 376(1) 2008: 1–12; doi:10.1016/j.ab.2007.12.012.
11 Ruhaak LR, et al. Hydrophilic Interaction Chromatography-Based High-Throughput Sample Preparation Method for N-Glycan Analysis from Total Human Plasma Glycoproteins. Anal. Chem. 80(15) 2008: 6119– 6126; doi:10.1021/ac800630x.
12 Varadi C, Lew C, Guttman A. Rapid Magnetic Bead Based Sample Preparation for Automated and High-Throughput N-Glycan Analysis of Therapeutic Antibodies. Anal. Chem. 86(12) 2014: 5682–5687; doi:10.1021/ac501573g.
13 Kornfeld S, Kornfeld R. Assembly of Asparagine-Linked Oligosaccharides. Annu. Rev. Biochem. 54, 1985: 631–664.
14 Hirschberg CB, Robbins PW, Abeijon C. Transporters of Nucleotide Sugars, ATP, and Nucleotide Sulfate in the Endoplasmic Reticulum and Golgi Apparatus. Annu. Rev. Biochem. 67, 1998: 49–69; doi:10.1146/annurev.biochem.67.1.49.
15 Kamiya Y, Satoh T, Kato K. Recent Advances in Glycoprotein Production for Structural Biology: Toward Tailored Design of Glycoforms. Curr. Opin. Struct. Biol. 26(1) 2014: 44–53; doi:10.1016/j.sbi.2014.03.008.
16 Gramer MJ, et al. Modulation of Antibody Galactosylation Through Feeding of Uridine, Manganese Chloride, and Galactose. Biotechnol. Bioeng. 108(7) 2011: 1591–1602; doi:10.1002/bit.23075.
17 Grainger RK, James DC. CHO Cell Line Specific Prediction and Control of Recombinant Monoclonal Antibody N-Glycosylation. Biotechnol. Bioeng. 110(11) 2013: 2970–2983; doi:10.1002/bit.24959.
18 Fan Y, et al. A Multipronged Investigation into the Effect of Glucose Starvation and Culture Duration on Fed-Batch CHO Cell Culture. Biotechnol. Bioeng. 112(10) 2015: 2172–2184; doi:10.1002/bit.25620.
19 Kildegaard HF, et al. Glycoprofiling Effects of Media Additives on IgG Produced by CHO Cells in Fed-Batch Reactors. Biotechnol. Bioeng. 113(2) 2016: 359–366; doi:10.1002/bit.25715.
20 Witsell DL, Casey CE, Neville MC. Divalent Cation Activation of Galactosyltransferase in Native Mammary Golgi Vesicles. J. Biol. Chem. 265(26) 1990: 15731–15737.
21 Yu X, et al. Engineering Hydrophobic Protein–Carbohydrate Interactions to Fine-Tune Monoclonal Antibodies. J. Am. Chem. Soc. 135(26) 2013: 9723–9732; doi:10.1021/ja4014375.
22 Barb AW, Prestegard JH. NMR Analysis Demonstrates Immunoglobulin G N-Glycans Are Accessible and Dynamic. Nat. Chem. Biol. 7(3) 2011: 147–153; doi:10.1038/nchembio.511.
23 Hsieh P, Rosner MR, Robbins PW. Host-Dependent Variation of Asparagine-Linked Oligosaccharides at Individual Glycosylation Sites of Sindbis Virus Glycoproteins. J. Biol. Chem. 258(4) 1983: 2548–2553.
24 Trimble RB, Maley F, Chu FK. Glycoprotein Biosynthesis in Yeast: Protein Conformation Affects Processing of High Mannose Oligosaccharides on Carboxypeptidase Y and Invertase. J. Biol. Chem. 258(4) 1983: 2562–2567.
25 Wormald MR, et al. Variations in Oligosaccharide — Protein Interactions in Immunoglobulin G Determine the Site-specific Glycosylation Profiles and Modulate the Dynamic Motion of the Fc Oligosaccharides. Biochem. 36(6) 1997: 1370–1380; doi:10.1021/bi9621472.
26 Fortunato ME, Colina CM. Effects of Galactosylation in Immunoglobulin G from All-Atom Molecular Dynamics Simulations. J. Phys. Chem. B 118(33) 2014: 9844–9851; doi:10.1021/jp504243e.
27 Barb AW, et al. NMR Characterization of Immunoglobulin G Fc Glycan Motion on Enzymatic Sialylation. Biochem. 51(22) 2012: 4618–4626; doi:10.1021/bi300319q.
28 Grey AA, et al. Structure of the Glycopeptides of a Human γ1-Immunoglobulin G (Tem) Myeloma Protein As Determined By 360-Megahertz Nuclear Magnetic Resonance Spectroscopy. Can. J. Biochem. 60(12) 1982: 1123–1131.
29 Rao AK, Mendicino J. Influence of Glycopeptide Structure on the Regulation of Galactosyltransferase Activity. Biochem. 17(26) 1978: 5632–5638.
30 Blanken WM, Hooghwinkel GJM, Van Den Eijnden DH. Biosynthesis of BloodGroup I and i Substances: Specificity of Bovine Colostrum β-N-Acetyl-d-Glucosaminide β1→4 Galactosyltransferase. Eur. J. Biochem. 127(3) 1982: 547–552.
31 Paquet MR, et al. Branch Specificity of Purified Rat Liver Golgi UDP-Galactose: N-Acetylglucosamine β-1,4-Galactosyltransferase — Preferential Transfer of Galactose on the GlcNAcβ1,2-Manα1,3-Branch of a Complex Biantennary Asn-Linked Oligosaccharide. J. Biol. Chem. 259(8) 1984: 4716–4721.
32 Wright A, et al. In Vivo Trafficking and Catabolism of IgG1 Antibodies with Fc Associated Carbohydrates of Differing Structure. Glycobiol. 10(12) 2000: 1347–1335.
33 Hodoniczky J, Zheng YZ, James DC. Control of Recombinant Monoclonal Antibody Effector Functions By Fc N-Glycan Remodeling In Vitro. Biotechnol. Prog. 21(6) 2005: 1644–1652; doi:10.1021/bp050228w.
34 Kochanowski N, et al. Influence of Intracellular Nucleotide and Nucleotide Sugar Contents on Recombinant Interferon-γ Glycosylation During Batch and Fed-Batch Cultures of CHO Cells. Biotechnol. Bioeng. 100(4) 2008: 721–733; doi:10.1002/bit.21816.
35 Anthony RM, et al. Recapitulation of IVIG Anti-Inflammatory Activity with a Recombinant IgG Fc. Science 320(5874) 2008: 373–376; doi:10.1126/science.1154315.
36 Karsten CM, et al. Anti-Inflammatory Activity of IgG1 Mediated By Fc Galactosylation and Association of FcγRIIB and Dectin 1. Nature Med. 18(9) 2012: 1401–1406; doi: 10.1038/nm.2862.
37 Jegouzo SAF, et al. A Novel Mechanism for Binding of Galactose-Terminated Glycans By the C-Type Carbohydrate Recognition Domain in Blood Dendritic Cell Antigen 2. J. Biol. Chem. 290(27) 2015: 16759–16771; doi:10.1074/jbc.M115.660613.
Further Reading
Bowden TA, et al. Chemical and Structural Analysis of an Antibody Folding Intermediate Trapped During Glycan Biosynthesis. J. Am. Chem. Soc. 134(42) 2012: 17554–17563; doi:10.1021/ja306068g.
Chen W, et al. Structural and Energetic Basis of Carbohydrate–Aromatic Packing Interactions in Proteins. J. Am. Chem. Soc. 135(26) 2013: 9877–9884; doi:10.1021/ja4040472.
Varadi C, et al. Analysis of Haptoglobin N-Glycome Alterations in Inflammatory and Malignant Lung Diseases By Capillary Electrophoresis. Electrophoresis 34(16) 2013: 2287–2294; doi:10.1002/elps.201300041.
Corresponding author Roger Anderson is a senior scientist, Lindsay Hock is a process development associate, and Sadettin S. Ozturk is deputy director for process development at MassBiologics, University of Massachusetts Medical School, 460 Walk Hill Street, Boston, MA 02126; 1-617-474-3000; www.umassmed.edu/Massbiologics. Rachel Yao is now a senior associate scientist at Unum Therapeutics (Cambridge, MA).