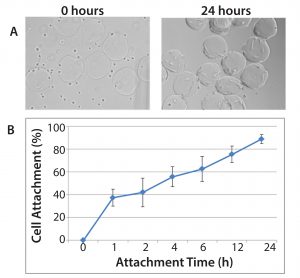
Figure 1: Demonstration of hMSC attachment to Corning Synthemax II–coated dissolvable microcarriers; (A) hMSCs and Synthemax II–coated dissolvable microcarriers during the cell attachment phase at 0 hours (left panel) and 24 hours (right panel). 10× magnification; (B) cell attachment efficiency for hMSCs on Synthemax II-coated dissolvable microcarriers (n = 3); cell attachment efficiency was determined by quantifying the number of unattached cells in the culture.
In recent years we have seen an exponential increase in the number of companies testing and validating new regenerative medicine products. Many of these products are reaching late-phase trials with the potential to receive final approval and marketing authorization from regulatory agencies such as the US Food and Drug Administration (FDA) and the European Medicines Agency (EMA). In the past several years, we have seen successful launches of regenerative medicine products, including Holoclar (Holostem Advanced Therapies), Kymriah (tisagenlecleucel, Novartis), Yescarta (axicabtagene ciloleucel, Kite Pharama), and Luxturna (voretigene neparvovec-rzyl, Spark Therapeutics).
With additional promising regenerative medicine products on the horizon, manufacturers have raised questions concerning process optimization and scalability. Many stem cells, such as human mesenchymal stem cells (hMSCs), can be readily cultivated in smaller flasks and laboratory-scale volume bioreactors. Because the dosage for clinical applications requires billions of hMSCs or anchorage-dependent cells, the challenge facing many regenerative medicine companies is the ability to commercially cost-effectively produce those cell quantities. Using multiple flasks to achieve a desired dosage would require significant investment in labor and increase the risk for variability and contamination that could arise from using numerous vessels. Therefore, an inability to cost-effectively scale up a process is a problem that can hinder further advances in regenerative medicine.
Microcarrier-based culture systems have long been thought of as the best technology to address the challenges of high-density stem cell culture for regenerative medicine. Microcarriers tend to be small beads (typically a diameter between 100 and 400 µm) that provide a high ratio of surface area to volume. They are made from inert materials such as polymers, dextran, or glass. The use of microcarriers in stirred-tank bioreactors can provide desired dosage requirements with higher cell yields. Cells grown using microcarriers can be expanded as one vessel or batch, thus reducing variability and contamination risks associated with multiple batches.
Although not all cell types can be readily expanded with microcarriers, a number of studies report successful scale-up of hMSCs on microcarriers in stirred-tank bioreactors (1, 2). These studies have shown the importance of selecting the appropriate microcarrier because factors such as material type, surface chemistry, cell seeding density, and medium composition can affect the outcome of a scale-up process. Researchers have voiced their concerns regarding microcarrier materials and surface chemistry because such components can significantly affect cell attachment and subsequent expansion. For instance, materials and surface chemistries that increase cell attachment of fastidious cells can cause problems during proliferation, expansion kinetics, and harvest. That can reduce overall cell yield and compromise phenotypic functionality of harvested cells.
To improve cell seeding and harvesting steps when working with stem cells — especially hMSCs grown on microcarriers in stirred-tank bioreactors — Corning has developed dissolvable microcarriers. They are made from cross-linked polysaccharide polymers and can be degraded effectively during cell harvest. Thus they provide a solution for large-scale expansion of hMSCs for therapeutic applications. The ability to dissolve microcarriers simplifies downstream purification processes compared with those for traditional microcarrers and eliminates the need to physically separate cells and microcarriers once a desired cell number is achieved.
Expansion of hMSC with Animal Component-Free Dissolvable Microcarriers
To demonstrate the feasibility of hMSC expansion on dissolvable microcarriers, we conducted a scale-up study using Synthemax II–coated dissolvable microcarriers. We cultured hMSCs for seven days in defined serum-free medium, expanded to cell yields exceeding 40,000 cells/cm2 (more than seven-fold expansion). Harvested cells were characterized for marker expression, karyotype, and the ability to differentiate into adipocytes, chondrocytes, and osteocytes.
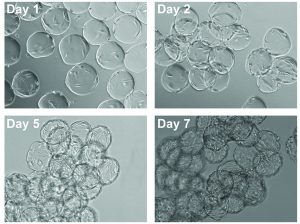
Figure 2: Images of cell expansion on Corning Synthemax II-coated dissolvable microcarriers; hMSCs on Synthemax II-coated dissolvable microcarriers on days 1, 2, 5, and 7 (10× magnification).
We monitored cell attachment to microcarriers for up to 24 hours after cell seeding (Figure 1). By the end of the attachment phase, nearly all cells were attached to the microcarriers, resulting in an attachment efficiency of 80–90%. Most important, the cells demonstrated uniform attachment to the microcarriers, with minimal bare microcarriers observed. The cells exhibited expected morphology after the attachment phase, suggesting that the microcarrier surface and cell attachment conditions were appropriate for hMSC culture.
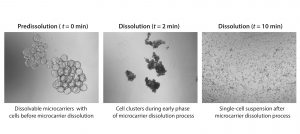
Figure 3: Microcarrier dissolution and cell release; images at different time points during the cell harvest phase (4× magnification); microcarriers were dissolved in a 1-L glass spinner flask following addition of a harvest solution consisting of pectinase, EDTA, and TrypLE. Microcarrier culture was mixed at 32 rpm during harvest phase.
Cells were expanded on the dissolvable microcarriers for seven days with half-volume media exchanges every two to three days. We observed a uniform distribution of cells for the first two to three days of the culture, with the cells continuing to demonstrate expected cell morphology (Figure 2). At the latter phase of the culture period, the cells achieved confluency.
We harvested cells on days 5, 6, and 7 of the expansion. Complete microcarrier dissolution was observed two to four minutes after addition of the harvest solution at all time points assessed, demonstrating the ease of dissolution of the dissolvable microcarriers (Figure 3).
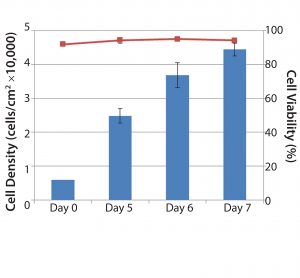
Figure 4: Quantitation of cell yield and cell viability following microcarrier dissolution and cell release; the entire microcarrier culture was harvested from individual 1 L glass spinner flasks on days 5, 6 and 7. Following microcarrier dissolution and cell release, cells were quantified on a Vi-CELL automated cell counter. Blue bars represent cell concentration (cells/cm2). Red line represents cell viability following cell harvest (n = 2, Days 5 and 6; n = 3, Day 7).
Results for overall cell yield from each culture following microcarrier dissolution and cell release showed that on day 5, cell concentrations reached 25,000 cells/cm2, corresponding to a fourfold expansion. By day 7, cell concentrations routinely exceeded 40,000 cells/cm2 (more than seven-fold expansion) with high viability (>90%) (Figure 4). Because the microcarriers can dissolve completely, cell recovery can improve significantly compared with results using other commercially available microcarriers.
We characterized hMSCs harvested from the dissolvable microcarriers fully for cell marker expression, karyotype, and the ability to differentiate into adipocyte, chondrocyte, and osteocyte cell lineages (Figure 5). We confirmed terminal differentiation into adipocyte, chondrocyte, and osteocyte lineages using traditional staining methods for characteristic markers. Flow cytometry confirmed the expression of positive surface markers CD73, CD90, and CD105 and the absence of CD14 and CD34. Chromosome analysis confirmed that the cells maintained normal karyotypes. Characterization results demonstrate that hMSCs expanded and harvested from dissolvable microcarriers maintain their phenotype, multipotency, and genetic stability.
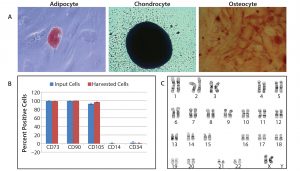
Figure 5: Characterization of hMSCs harvested from Corning Synthemax II–coated dissolvable microcarriers; cells harvested from dissolvable microcarriers were characterized for ability to differentiate into adipocytes, chondrocytes, and osteocytes; for expression of cell surface markers; and for genetic stability. (A) Confirmation of terminal differentiation of into adipocyte, chondrocyte, and osteocyte cell lineages followed incubation with an appropriate differentiation medium for 14–21 days. Cell differentiation was confirmed by staining with Oil Red O (adipocyte), Alcian Blue (chondrocyte), and Alizarin Red S (osteocyte). Adipocyte (20× magnification), chondrocyte, and osteocyte (2.5× magnification); (B) flow cytometry data for specific cell surface markers (n = 2); input cells represent cells used to seed dissolvable microcarriers. (C) Demonstration of normal karyotype for hMSCs harvested from dissolvable microcarriers; live cultures were submitted for G-banded karyotype analysis
A Scalable Platform for Cell Expansion
Dissolvable microcarriers provide a scalable solution for large-scale expansion and harvest of functional hMSCs by enabling simplified downstream processing and high-yield cell recovery. We observed complete dissolution of the microcarriers during the harvest phase, which resulted in high recovery of functional hMSCs. The harvested cells maintained multipotency and demonstrated expected phenotype.
The emergence of regenerative medicine and cell-based therapies is driving demand for large quantities of high-quality cells to support clinical trials and commercial availability. Existing approaches for culturing those cells have limitations that hamper their ability to generate sufficient quantities of therapeutically active cells using well-controlled processes. Novel approaches such as the dissolvable microcarriers described above will help industrialize cell therapy development.
Acknowledgment
We acknowledge Hannah Gitschier and Christopher Suarez for their valuable input.
References
1 Tsai AC, Ma T. Expansion of Human Mesenchymal Stem Cells in a Microcarrier Bioreactor. Methods Mol. Biol. 1502, 2016: 77–86; doi:10.1007/7651_2016_338.
2 Panchalingam KM, et al. Bioprocessing Strategies for the Large-Scale Production of Human Mesenchymal Stem Cells: A Review. Stem Cell Res. Ther. 6, 2015: 225; doi: 10.1186/s13287-015-0228-5.
Dr. John Yoshi Shyu (shyujy@corning.com) currently oversees the field application scientist team in North America at Corning Life Sciences. Jessica L. Martin (martinJ2@corning.com) is bioprocess product line manager at Corning Life Sciences.