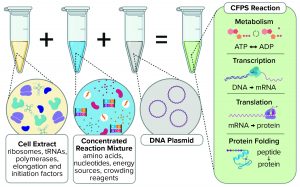
Components of a cell-free protein synthesis reaction (extract, supplements, and a DNA template) with the key reactions that occur when they are combined
Cell-free synthesis (CFS), also known as cell-free transcription and translation, supplements cellular components (either a cell lysate or purified recombinant elements) with nucleotides, amino acids, metabolic intermediates, and salts to produce a nucleic acid or protein from a genetic template added to the reaction. This exciting technology has seen a substantial increase in both academic and commercial interest over the past decade (1). Interest stems in large part from the potential to democratize access to the machinery of biology by removing the need to engineer cells genetically (2). CFS has the potential to revolutionize healthcare in much the same way that personal computers did for information technology (3). However, our interest and that of the bioprocessing community at large comes from the potential to transform manufacturing for some healthcare products (4).
A growing area in which CFS platforms are recognized as a potentially enabling technology is in stratified approaches to facilitate distributed manufacturing of biological products (5). Although still developing as a manufacturing technology, CFS offers flexibility and potentially improved robustness over existing cell-based biotherapeutic manufacturing. The technology could reduce on-site footprints and infrastructure complexity and allow for robust process control combined with flexibility of output. In this context, University College London’s biochemical engineering department — working with the Future Targeted Healthcare Manufacturing (FTHM) Hub supported by the United Kingdom’s Engineering and Physical Sciences Research Council (EPSRC) — has undertaken a series of workshops (pictured herein). They focused on elucidating drivers and barriers to the use of this technology for stratified medicines manufacture and mapping out desirable future states of the technology.
Here we summarize the FTHM Hub specialist working group’s discussions toward a roadmap for CFS platforms. We begin with a short history of CFS before proceeding to consider the motivations for commercial interest by looking at existing applications of the technology. Finally, we examine the technical challenges in applying CFS in bioprocessing and map the developmental stages toward a CFS-based device for distributed manufacturing.
A Brief History
CFS is not a new technique. It was used first in 1961 and played an important role in understanding the genetic code and central dogma of modern biology: the link between DNA, messenger RNA, and protein expression (6). Figure 1b illustrates this history with a plot of the number of papers related to CFS showing a peak of interest in the early 1970s, followed by a substantial revival of interest over the past two decades. CFS activity follows the features of a classical hype curve for a new technology (7). As Figure 1a also shows, the number of extract sources and product types has expanded rapidly, with CFS used for the production of antigens, virus-like particles (VLPs), cytokines, antibodies, peptides, membrane proteins, viable bacteriophages and viruses, enzymes containing metal cofactors, proteins containing nonstandard amino acids (also known as nonnatural amino acids), and RNA. Applications also have expanded rapidly, including advancements in mobile biosensors (8) and manufacturing platforms (5).
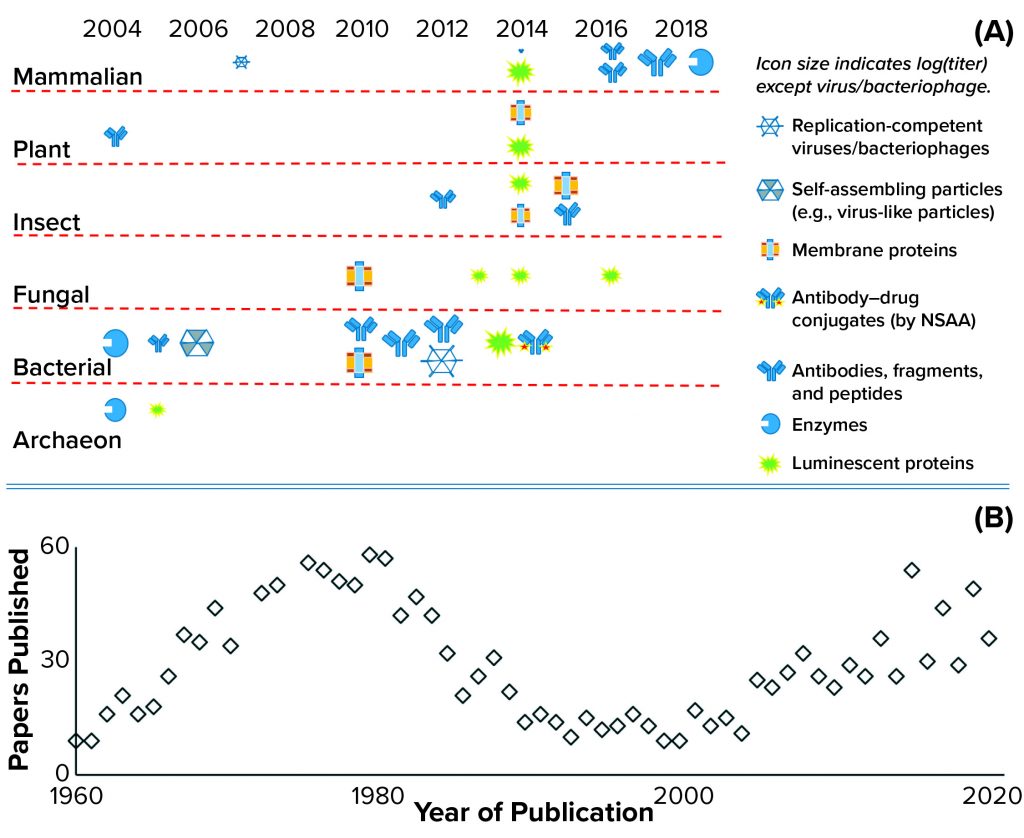
Figure 1: (a) Historic achievements in cell-free synthesis (CFS) across different cell types, with an indicative range of protein types and relative titers (log proportional to the icon size) achieved to date; the key achievement of incorporating nonstandard amino acids (NSAA) is highlighted. Another key achievement was cell-free glycosylation using Chinese hamster ovary (CHO) cell lysate in 2014. Bacterial extracts come from A19, Rosetta (DE3), BL21 (DE3), BL21, star (DE3), and ClearColi BL21 Escherichia coli strains that have been engineered to make them more suitable for manufacturing. Yeast extracts come from Pichia pastoris and Saccharomyces cerevisiae strains; mammalian extracts from CHO, human embryonic kidney (HEK), human epithelioid cervix carcinoma (HeLa) cells, and from blood-derived leukocytes; and plant extracts from wheat germ and tobacco. Results presented herein come from a range of research groups with limited or no standardization, so parameters such as the CFS reactor type (batch or substrate addition/inhibitor removal by dialysis), reaction mix and length do not match (see 13 and Further Reading). (b) The numbers of CFS papers published per year are based on a Scopus search for the terms cell-free and synthesis in article titles.
Research to date has focused largely on optimization of the CFS reaction mix (nucleotides, amino acids, metabolic intermediates, and salts) and on cell-extract production, particularly source-strain modifications and use of different cell types. Those efforts have produced order-of-magnitude improvements in titer, with 2.3 mg/mL the maximum cited so far (9).
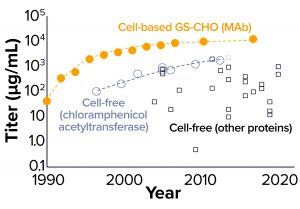
Figure 2: Comparing the evolution of titer in glutamine synthetase (GS) selected Chinese hamster ovary (CHO) cells and cell-free production systems (12, 13, and Further Reading)
Figure 2 compares the rate of titer improvement achieved with the Chinese hamster ovary (CHO) manufacturing cell line with that achieved in CFS systems. This comparison brings us to a number of key observations. First, the titers currently achievable in a CHO-based system are an order of magnitude greater than those obtainable with CFS (10) — shown to be comparable from both Escherichia coli and CHO CFS systems in Figure 1a. However, that should be considered in the context of very differing levels of investment. Intensive process development for the CHO system has been ongoing for decades. By way of comparison, the number of papers published in CFS has reached about 40 per year, whereas for CHO it is about 200 per year and has been increasing linearly since the early 1970s.
Second, titers reported from CFS reactions show a wide scatter, reflecting the diversity of products and platforms (cell types) involved. In addition, titer variation may have been exacerbated by a lack of cross-laboratory good practice for reaction conditions used (11) and the fact that few researchers in the domain are focused on producing their protein of interest for a preparative or manufacturing application. In 2012, Carlson et al. (12) presented data on the trend in titers for chloramphenicol acetyltransferase (CAT) produced in CFS reactions, which represent the most complete set of data yet available charting historic improvements in cell-free titers for a single recombinant protein product. If we consider that trend, then progress in CFS titers has been substantial and sustained, and it compares well with the trajectory achieved so far with monoclonal antibody (MAb) expression in CHO cells. That could reflect the openness of the system and relative simplicity and speed with which CFS process modifications can be implemented.
Considering both the rapid rate of titer improvements achieved to date and the demonstrated scalability of the technology (13), the potential of CFS is clear. So why is adoption of the technology limited to so few companies in this domain to date, and what applications for CFS could drive growth (11)? To answer these questions, we begin with a few exemplar companies and their rationale for using CFS.
Exemplar Commercial Bioprocess Applications
Antibody–Drug Conjugates (ADCs): Worldwide more than 50 ADCs currently are in clinical trials (14), and 250 are under development, with the market projected to be worth US$15 billion by 2030 (15). ADC product critical quality attributes (CQAs) often are more complex than those for therapeutic proteins alone, including attributes such as the ratio of conjugated drug to antibody (16). CFS offers the ability to incorporate nonstandard amino acids (NSAAs), which enables control of the location and number of conjugation sites (17), rapid prototyping of proteins, and subsequent scaling and incorporation of potent toxins as loads.
Based in South San Francisco, Sutro Biopharma was founded in 2003 and employs about 200 people. Its good manufacturing practice (GMP) production facilities use the proprietary Xpress CF+ cell-free platform to produce ADCs, bispecific antibodies, and cytokine-based therapies. The company’s pipeline includes drugs in clinical trial stages from discovery to phase 1 that have been developed alone and in partnerships with Bristol-Myers Squibb, EMD Serono, and Merck using Sutro’s platform (18).
Toxin Manufacture: Ipsen Biopharm is a global biopharmaceutical company with products in neuroscience, consumer healthcare, oncology, and rare diseases. One of its products is botulinum toxin used as a therapeutic for disorders caused by over-activity of muscles (19). The toxin is highly potent, so very low doses are required, and manufacturing is performed with relatively small-scale equipment. However, employees face substantial hazards during production (unusual for a biotherapeutic product) because of the extreme toxicity of the product. The challenge of managing those risks motivated this company’s interest in CFS. The absence of cells allows for relatively little manual intervention during toxin synthesis, thus reducing the risk of containment loss. A collaboration among Ipsen Biopharm, Touchlight Genetics (which has a cell-free DNA synthesis technology), and the UK Centre for Process Innovation (CPI) is under way with the goal of developing a fully enclosed process for safely and securely producing this toxin (20).
RNA Products: Headquartered in Medford, MA, GreenLight Biosciences was founded in 2009 and employs about 100 people. The company produces RNA using the proprietary GreenWorX cell-free platform. The company’s interests are in vaccine development, pandemic preparedness, and plant protection against disease and pests. Thus, the greater speed of response and lower cost compared with other methods for RNA production are key motivators for GreenLight to use CFS. Using RNA as an insecticide/fungicide is a novel technology that requires development (21), so the short prototyping turnover also is important. This company has partnerships with Bayer Crop Science, AgroSpheres, and Advanced BioNutrition.
Drivers for CFS Use
The case studies above show that uptake of CFS is driven by potential for
- improved control of product quality through standardized bulk manufacture of reagents as well as direct access to cellular machinery and mechanisms for protein engineering (e.g., introduction of NSAAs)
- the ability to make products that are difficult/impossible to express or make consistently using existing technology through removal of the need to keep cells alive and healthy, which broadens the available range of products, reaction conditions, and mechanisms for protein engineering
- ease of containment because of simplified equipment requirements in the absence of fermentation/cell culture and live genetically modified organisms
- increased speed of process development, enabling rapid-response manufacturing through shortened timelines for prototyping and subsequent scale-up (typical protein synthesis in a CFS system requires three to four hours rather than three to four weeks for CHO cell culture and two to three days for E. coli fermentation, excluding stable cell-line generation)
- increasing interest in mRNA-based therapeutics (22, 23) for which CFS/in vitro transcription is a leading manufacturing platform.
Within the context of the FTHM Hub, we were interested particularly in the applicability of CFS to personalized or stratified medicine. Intended to serve particular groups of patients identified by genetic screening and diagnostics, these drugs represent a more efficient use of limited resources and a better outcomes than might be available through the traditional model. It is anticipated that growing consumer knowledge and demand will increase the “market pull†for such medicines. That pull will come from both healthcare providers/funders and patients (24).
To enable stratified CFS production of therapeutic proteins/nucleic acids, many experts envision centralized large-scale production of raw materials and localized production of the final therapeutic product, as outlined by Ogonah et al. in 2017 (4). In principle, CFS is well suited for such localized manufacturing because of the reduced infrastructure and expertise required for protein production, making it suitable for automation and amenable to reproducibility/predictability improvements over the current biomanufacturing model.
To date, cost of goods (CoG) reduction has not been a primary driving factor for the uptake of CFS, and it is unlikely to be so in the future. Indeed, analysis conducted within the FTHM Hub suggests that prokaryotic-based CFS currently is more expensive ($/mg) than CHO-cell–based processes, although only by a factor of about two (25). With protein biologics designed for small groups of patients, the route to reduced costs no longer can come from economies of scale, no matter the platform, which gives further impetus to reexamining our manufacturing paradigms. Some evidence suggests that at personalized or orphan-medicine scales, the economics may favor CFS (10). With its raw material components produced at scale, the goal can be minimal customization from product to product. It also is anticipated that costs will decrease substantially over time — e.g., by use of modified or alternative source strains (26, 27), with cell extracts currently representing a substantial proportion of the overall costs (25).
Challenges to CFS Uptake
Although the development rate for this technology is a function of both commercial and technical factors (24), here we focus only on the technical and regulatory barriers to uptake. First we consider the possibility that the same technical outcomes can be achieved through a competing technology. Then we assess the size of the technical obstacles to be overcome for arriving at a CFS-based device that will be acceptable to regulators for distributed manufacturing.
Competing Technologies for Stratified Medicines Production: Cell-based synthesis has been and continues to be the highly effective format of choice for most companies making or wishing to make recombinant proteins. We do not anticipate CFS to supplant cell-based manufacture entirely. However, as highlighted above and summarized in Table 1, CFS does offer a number of distinct advantages, particularly for distributed manufacturing. The costs, time, and resources required for development of a larger range of treatments, each with a naturally limited market, imply that stratified medicines will require streamlined development and manufacture. But can existing technologies or alternatives in development match CFS?
Such alternative approaches include
- decentralized manufacture using the currently predominant cell-based batch manufacturing
- intensified cell-based manufacture (e.g., continuous bioprocessing (28, 29))
- modular manufacturing units (30)
- use of alternative host organisms with simplified requirements (31–33)
- use of transient transfection approaches (10).
Within cell-based production, only intensified production with alternative hosts offers the possibility of relatively simple automation (without expensive robotics for handling cell culture processes/equipment) that might enable localized production of biotherapeutics (33). However, the complexity of the equipment and expertise required to control for variability in cellular responses must be resolved to achieve full automation (34, 35). For cell-based systems, maintenance of cell banks also will remain necessary, complicating distribution and storage of reagents.
A continuous production platform based on Pichia pastoris, an expression system capable of product secretion with low levels of harvest host-cell proteins (HCPs), has been proposed and trialed under the auspices of the Biologically-Derived Medicines on Demand (Bio-MOD) project of the US Defense Advanced Research Projects Agency (DARPA) (33). The bio-MOD program also funded a CFS-based tool for “battle-field medicine†(36). Considerable efforts have gone into engineering human-like glycosylation in the P. pastoris host, but it has yet to be fully resolved (37). Therefore, similar technologies also are in development using mammalian cells, which do require longer timelines with a more complex host cell but also offer an established method for direct human-like glycosylation of protein products. It could be argued that host types that produce aglycosylated proteins, either in cell-free or cell-based systems, might enable greater product quality control through subsequent enzymatic glycosylation (38).
Other technologies exist or are in development for modification of cell-lines and acceleration of cell-line development for biotherapeutics. However, even at an accelerated rate, cell-line development and cell culture cannot match the development and production timelines achieved with CFS. It should be acknowledged that currently the CoG and (crude lysate) prepurification HCP/DNA levels would be higher for material produced in a CFS platform than for the proposed P. pastoris–based continuous platform. Nonetheless the potential of CFS remains clear set against its competitors.
Identifying Key Technical Hurdles: Having established an understanding of the current landscape and potential for cell-free technology, the FTHM Hub workshop team developed a problem statement. Its purpose was to help elucidate what would be required from a CFS platform to make it a viable manufacturing technology for stratified medicine. Here is the statement agreed upon: “What are the technical challenges to making an injectable biotherapeutic without cells, using automated, on-demand, localized manufacture?†Figure 3 summarizes the outcomes of this exercise.
As highlighted by the red boxes in Figure 3, the concerns of the industrial partners with an interest in CFS largely related to quality control and regulatory release. The challenge for CFS in this respect relates to
- complexity of the raw material (particularly cell extract) and characterization of its CQAs
- limited understanding of which raw-material attributes will influence reaction performance, which is plasmid/product-specific and therefore requires a process development strategy
- a need to establish appropriate in-process monitoring technologies
- a quality by design (QbD) approach to validation for the device to enable drug-substance release (39).
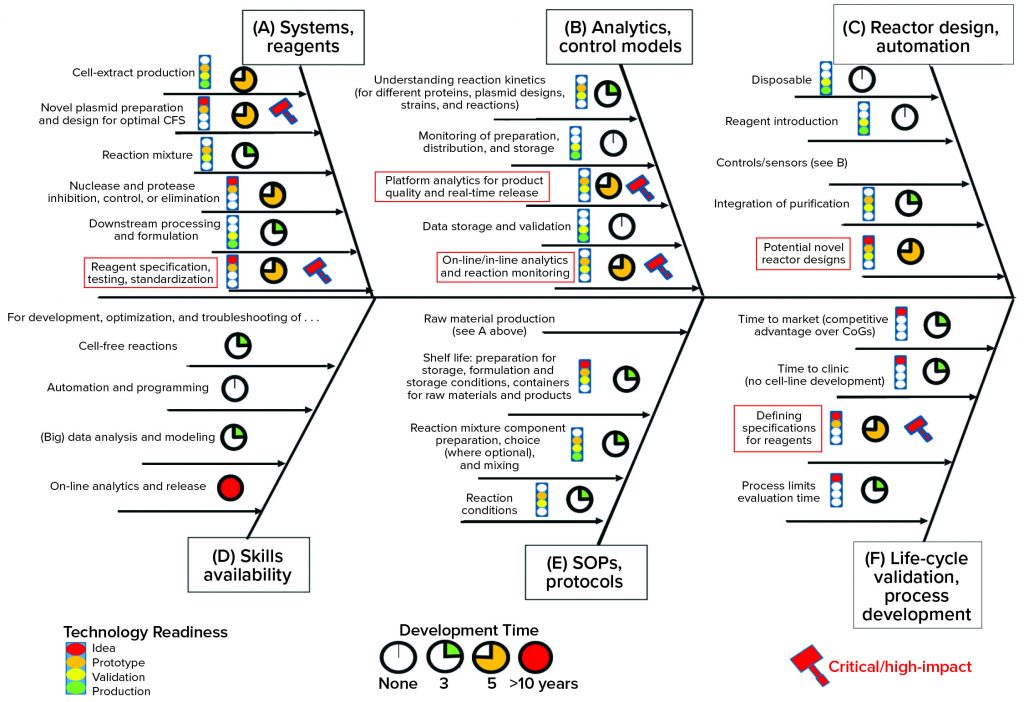
Figure 3: This Ishikawa (“fishboneâ€) diagram shows technical, organizational, and logistical challenges in achieving the objective of “making an injectable biotherapeutic without cells, using automated, on-demand, localized manufacture,†as identified by the academic and industrial participants in the FTHM Hub (see Acknowledgments). Red boxes highlight critically important areas. An intermediate phase of technology readiness is indicated by more than one color in the traffic-light icons (11, Further Reading).
The importance of cell extract as a raw material is highlighted by the fact that two companies discussed in the case studies above stress their use of in-house developed and proprietary extracts and protocols. Furthermore, published literature dealing with the question of extract preparation is extensive (although less so at the industrial scale) but diverse and still moving toward an accepted best practice (40). Even at laboratory scale, the sensitivity of cell-extract qualities to the production process is poorly understood, and such processes require systematization and rationalization to achieve robustness (11, 41). This issue was recently referred to by the CPI, which claims to have developed a scalable and simplified process for lysate production to address the issue (42).
After production of raw materials, CFS-reaction robustness and the connection between measured process parameters and final-product quality will need to be understood and ensured. The expectation is that tight control of raw-material attributes and characterization of the reaction’s response to critical process parameters (CPPs, e.g., temperature and pH), will bring cell-free reactions close to the reproducibility seen in many small-molecule and enzymatic processes (without undue influence of stochastic or unidentified elements). To gather such understanding will require a combination of high-throughput experiments, for which CFS reactions are well suited, with appropriate modeling and control (43), such as through hybrid metabolic models like those proposed for cellular systems (44).
Finally, plasmid preparation (10, 11) and design (45, 46) are fields suggested by published literature and our own experience as likely to have a large impact on CFS titers.
Toward the RoadmapÂ
Figure 4 shows a series of prototypes for a compact, localized CFS platform. On the left, the first prototype is based on systems described by Adiga et al. (5), Pardee et al. (47), and Crowell et al. (33). The latter developed a system using continuous cell-based production, however that overlaps with technology developments required for stratified processing, whether cell-based or cell-free, such that both technologies can be informed by each other (43). And recently reported collaborative work between CureVac and Tesla to generate an automated mRNA production platform also represents a technology from which a CFS platform could draw (48, 49).
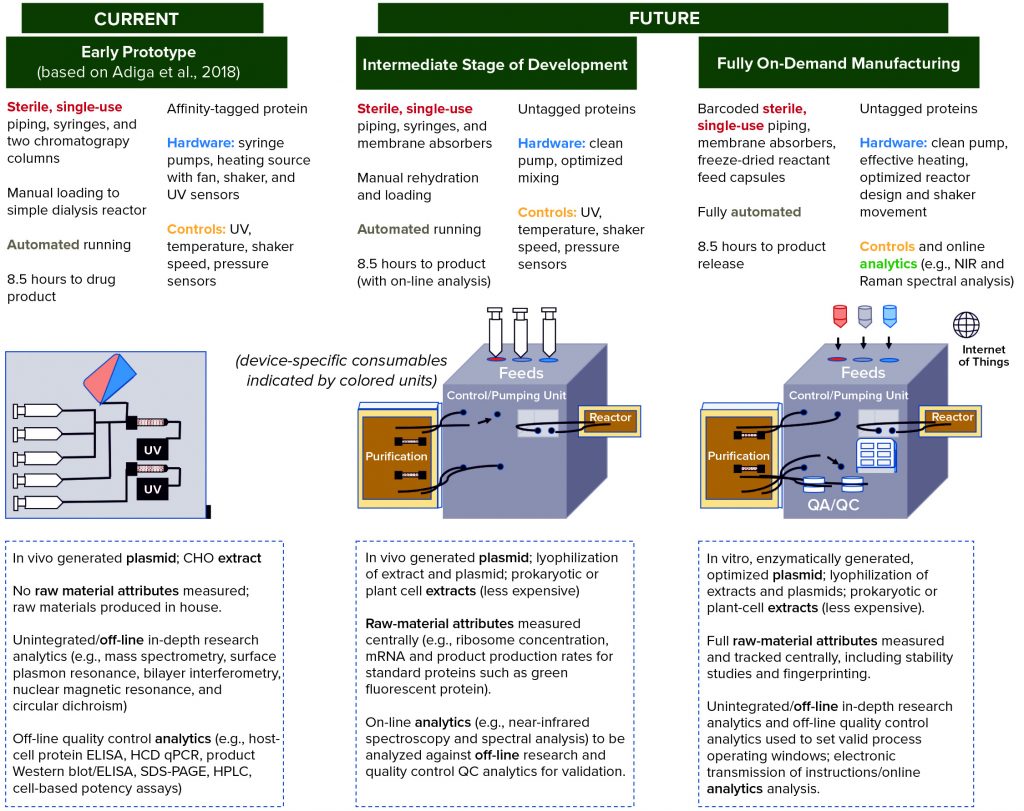
Figure 4: Roadmap with development stages of prototype devices envisioned to achieve production of “an injectable biotherapeutic without cells, using automated, on-demand, localized manufacture,†starting with a system based on that described by Adiga et al. (5), with additions from Pardee et al. (47) and Crowell et al. (33). Subsequent intermediate prototype and fully on-demand manufacturing are based on the technical development areas identified in Figure 3 and our understanding of which ones can and need to be addressed first. Device schematics and descriptions indicate distributed elements of the technology. Text below the schematics (in dashed boxes) indicates raw material and quality control (QC) development activities that might be completed elsewhere (e.g., at a centralized facility). Green text indicates the first appearance of a feature retained in the final device.
Figure 4 also clarifies the importance of supporting technologies, particularly related to the centralized supply of tightly controlled raw materials and a system for on-board product quality measurement, control, and release. Centralized raw-material production will enable investment in robustness and reproducibility for economies of scale, with specifications set independently of a specific product and development of analytical techniques aided by consistency in raw materials.
Growing scientific understanding of disease pathology and the individuality of the treatment responses brings great potential for a revolution in biotherapeutic efficacy and safety. However, that will require a new paradigm in biotherapeutic production to sit in parallel with existing biomanufacturing of blockbuster drugs. The speed of development for this technology will depend on multiple factors — e.g., government interest/support, identification of early commercial applications, and vested interests in established technologies (24) — in order to secure sufficient investment to overcome the technical challenges highlighted herein. These technical challenges are considerable, as is the coordination and cross-disciplinary working that will be required to overcome them. But the history of MAb processes based on CHO cells shows what can be achieved by a concerted effort when the potential of a technology is recognized. We hope that our analysis will inspire further debate, collaboration, and research toward realizing the potential of CFS in biomanufacturing.
Acknowledgments
We gratefully acknowledge funding from the UK Engineering and Physical Sciences Research Council (EPSRC) for the Future Targeted Healthcare Manufacturing Hub hosted at University College London (UCL) with UK university partners (grant EP/P006485/1). We also acknowledge financial and in-kind support from the consortium of industrial users and sector organizations.
We received input from attendees at the Cell-Free Protein Synthesis Specialized Working Group within UCL’s FTHM Hub: Marc-Olivier Baradez (Cell and Gene Therapy Catapult); Harvey Branton and Philip Probert (Centre for Process Innovation); Stuart Hassard (deltaDOT); Andy Topping (Fujifilm Diosynth Biotechnologies); Daniele Perna (GlaxoSmithKline); Rochelle Aw, Chiara Heide, Alex Spice (Imperial College London); David Gruber (Ipsen Biopharm); Marcel Kuiper (Knowledge Transfer Network); Andy Racher and Jesus Zurdo (Lonza); John Welsh (Pall); Martin Smyth (Sartorius Stedim Biotech); Nick Major (Sekisui Diagnostics); Trevor Hallam (Sutro Biopharma); Paul Dalby, Olotu Ogonah, Jamie Teneb-Lobos, Stefanie Frank, Darren Nesbeth, and Chileab Redwood-Sawyer (UCL); and Carlos-Alberto Duran-Villalobos and Barry Lennox (University of Manchester). Thanks also to Eleanor Bonnist (UCL) for her help in organizing these meetings.
References
1 Dopp BJL, Tamiev DD, Reuel NF. Cell-Free Supplement Mixtures: Elucidating the History and Biochemical Utility of Additives Used to Support In Vitro Protein Synthesis in E. coli Extract. Biotechnol. Adv. 37(1) 2019: 246–258.
2 Costa K, Hyde E. Cell-Free Expression Platforms Enable New Possibilities at iGEM and Beyond. SynBioBeta 29 November 2018; https://synbiobeta.com/cell-free-expression-platforms-enable-new-possibilities-at-igem-and-beyond.
3 Pardee K. Perspectives: Solidifying the Impact of Cell-Free Synthetic Biology Through Lyophilization. Biochem. Eng. J. 138, 15 October 2018: 91–97.
4 Ogonah OW, Polizzi KM, Bracewell DG. Cell Free Protein Synthesis: A Viable Option for Stratified Medicines Manufacturing? Curr. Opin. Chem. Eng. 18, 2017: 77–83.
5 Adiga R, et al. Point-of-Care Production of Therapeutic Proteins of Good-Manufacturing-Practice Quality. Nat. Biomed. Eng. 2(9) 2018: 675–686.
6 Nirenberg M, Matthaie J. The Dependence of Cell-Free Protein Synthesis in E. coli upon Naturally Occurring or Synthetic Polyribonucleotides. Proc. Nat. Acad. Sci. USA 47(10) 1961: 1588–1602.
7 Fenn J, Raskino M. Mastering the Hype Cycle: How to Choose the Right Innovation at the Right Time. Harvard Business Press: Boston, MA, 2008.
8 Paper-Based Diagnostics. Wyss Institute: Boston, MA, 2020; https://wyss.harvard.edu/technology/paper-based-diagnostics/.
9 Caschera F, Noireaux V. Synthesis of 2.3 mg/mL of Protein with an all Escherichia coli Cell-Free Transcription-Translation System. Biochimie 99, 2014: 162–168.
10 Thaore V, et al. Techno-Economic Assessment of Cell-Free Synthesis of Monoclonal Antibodies Using CHO Cell Extracts. Processes 8(4) 2020: 454–468.
11 Romantseva E, Strychalski EA. CELL-FREE (Comparable Engineered Living Lysates for Research Education and Entrepreneurship) Workshop Report. NIST Special Publication 1500-13. National Institute of Standards and Technology: Gaithersburg, MD, 2020.
12 Carlson ED, et al. Cell-Free Protein Synthesis: Applications Come of Age, Supplementary Data. Biotechnol. Adv. 30, 2012: 1185–1194.
13 Zawada JF, et al. Microscale to Manufacturing Scale-Up of Cell-Free Cytokine Production: A New Approach for Shortening Protein Production Development Timelines. Biotechnol. Bioeng. 108(7) 2011: 1570–1578.
14 Report ID: GVR-2-68038-741-4. Antibody Drug Conjugates Market Size, Share and Trends Analysis Report by Application (Brain Tumor, Blood, Breast, Ovarian, Lung Cancer), by Technology (Cleavable, Non-Cleavable Linker), and Segment Forecasts, 2019–2025. Grand View Research, Inc.: San Francisco, CA, January 2019.
15 Chaudhary G. The Antibody Drug Conjugates Market Is Projected to Be Worth More than USD 15 Billion by 2030, Growing at a CAGR of Over 20%. Bloomberg 29 August 2019.
16 Swarmy J, McDermott L. Expert View: What ADC Evolution Means for Manufacturing. Eur. Pharm. Rev. 19 December 2018.
17 Zimmerman ES, et al. Production of Site-Specific Antibody-Drug Conjugates Using Optimized Non-Natural Amino Acids in a Cell-Free Expression System. Bioconjug. Chem. 25(2) 2014: 351–361.
18 Pipeline. Sutro Biopharma: South San Francisco, CA, 2020; https://www.sutrobio.com/pipeline.
19 Munchau A, Bhatia KP. Uses of Botulinum Toxin Injection in Medicine Today. BMJ 320(772) 2020: 161–165.
20 Ipsen Biopharm Ltd. Novel Production Process for a Highly Potent Recombinant Protein Using Doggybone DNA (dbDNA) Vector and Cell Free Expression Technology. UK Research and Innovation 2020; https://gtr.ukri.org/projects?ref=104201.
21 Maxwell B, et al. Enabling the RNA Revolution: Cell-Free dsRNA Production and Control of Colorado Potato Beetle. GreenLight Biosciences: Medford, MA, 2019.
22 Foster JB, Barrett DM, Kariko K. The Emerging Role of In Vitro-Transcribed mRNA in Adoptive T Cell Immunotherapy. Mol. Ther. 27(4) 2019: 747–756.
23 Stanton D. One Billion Doses: CureVac Gains $88m to Support Capacity for COVID-19 mRNA Vaccine. BioProcess Insider 18 March 2020.
24 Clarke LJ. Synthetic Biology: Pathways to Commercialisation. Eng. Biol. 3(1) 2019: 2–5.
25 Stamatis C, Farid S. Process Economics Evaluation of Cell-Free Synthesis for the Commercial Manufacture of Antibody Drug Conjugates. Submitted 2020.
26 Buntru M, et al. Tobacco BY-2 Cell-Free Lysate: An Alternative and Highly-Productive Plant-Based In Vitro Translation System. BMC Biotechnol. 14(37) 2014.
27 Buntru M, et al. A Versatile Coupled Cell-Free Transcription-Translation System Based on Tobacco BY-2 Cell Lysates. Biotechnol. Bioeng. 112(5) 2015: 867–878.
28 Alper J, et al. Continuous Manufacturing for the Modernization of Pharmaceutical Production: Proceedings of a Workshop. National Academies Press: Washington, DC, 2019.
29 Hajba L, Guttman A. Continuous-Flow-Based Microfluidic Systems for Therapeutic Monoclonal Antibody Production and Organ-on-a-Chip Drug Testing. J. Flow Chem. 7(3–4) 2017: 118–123.
30 Hernandez R. Modular Manufacturing Platforms for Biologics. BioPharm Int. 28(5) 2015: 18–25.
31 Jiang H, et al. Challenging the Workhorse: Comparative Analysis of Eukaryotic Micro-Organisms for Expressing Monoclonal Antibodies. Biotechnol. Bioeng. 116(6) 2019: 1449–1462.
32 Love KR, Dalvie NC, Love JC. The Yeast Stands Alone: The Future of Protein Biologic Production. Curr. Opin. Biotechnol. 53, 2018: 50–58.
33 Crowell LE, et al. On-Demand Manufacturing of Clinical-Quality Biopharmaceuticals. Nat. Biotechnol. 1 October 2018.
34 Silverman AD, Karim AS, Jewett MC. Cell-Free Gene Expression: An Expanded Repertoire of Applications. Nat. Rev. Genet. 21(3) 2020: 151–170.
36 Arnaud C. Making Biologics on Demand. Chem. Eng. News 96(45) 2018.
37 Beck A, Cochet O, Wurch T. GlycoFi’s Technology to Control the Glycosylation of Recombinant Therapeutic Proteins. Exp. Opin. Drug Discov. 5(1) 2010: 95–111.
38 In Vitro Glycoengineering of Therapeutic Proteins. Roche Diagnostics International: Risch-Rotkreuz, Switzerland, 2020; https://custombiotech.roche.com/home/applications/in-vitro-glycoengineering—take-control-of-glycosylation.html.
39 Gingery D. Individualized Gene Therapy: FDA Considering Device-Like Manufacturing Approval Process. BioProcess Insider 9 March 2020.
40 Dopp JL, Jo YR, Reuel NF. Methods to Reduce Variability in E. coli–Based Cell-Free Protein Expression Experiments. Synth. Syst. Biotechnol. 4(4) 2019: 204–211.
41 Spirin AS, Swartz JR. Cell-Free Protein Synthesis Systems: Historical Landmarks, Classification, and General Methods. Cell-Free Protein Synthesis. Spirin AS, Swartz JR, Eds. Wiley VCH: Weinheim, Germany, 2008.
42 Macdonald GJ. Cell Out? Lysate-Based Expression an Option for Personalized Meds. Gen. Eng. News 18 February 2020.
43 Hong MS, et al. Challenges and Opportunities in Biopharmaceutical Manufacturing Control. Comput. Chem. Eng. 110, 2018: 106–114.
44 Richelle A, et al. Towards a Widespread Adoption of Metabolic Modeling Tools in Biopharmaceutical Industry: A Process Systems Biology Engineering Perspective. NPJ Syst. Biol. Appl. 6(6) 2020: 6.
45 Abe N, et al. Rolling Circle Amplification in a Prokaryotic Translation System Using Small Circular RNA. Angew Chem. Int. Ed. Engl. 52(27) 2013: 7004–7008.
46 Thoring L, et al. Cell-Free Systems Based on CHO Cell Lysates: Optimization Strategies, Synthesis of “Difficult-to-Express†Proteins and Future Perspectives. PLoS One 11(9) 2016: e0163670.
47 Pardee K, et al. Portable, On-Demand Biomolecular Manufacturing. Cell 167(1) 2016: 248–259 e12.
48 Macdonald G. Instant caRNA? Tesla Touts RNA Bioreactor It Is Developing with Curevac. BioProcess Insider 6 July 2020.
49 Delbert C. Tesla Has Been Working on an RNA Bioreactor. Pop. Mechan. 6 July 2020.
Figures: Further Reading
Ali M, et al. Improvements in the Cell-Free Production of Functional Antibodies Using Cell Extract from Protease-Deficient Escherichia coli Mutant. J. Biosci. Bioeng. 99(2) 2005: 181–186.
Brodel AK, Sonnabend A, Kubick S. Cell-Free Protein Expression Based on Extracts from CHO Cells. Biotechnol. Bioeng. 111(1) 2014: 25–36.
Burgenson D, et al. Rapid Recombinant Protein Expression in Cell-Free Extracts from Human Blood. Sci. Rep. 8(1) 2018: 9569.
Endoh T, Kanai T, Imanaka T. A Highly Productive System for Cell-Free Protein Synthesis Using a Lysate of the Hyperthermophilic Archaeon, Thermococcus kodakaraensis. Appl. Microbiol. Biotechnol. 74(5) 2007: 1153–1161.
Focke PJ, et al. Combining In Vitro Folding with Cell Free Protein Synthesis for Membrane Protein Expression. Biochemistry 55(30) 2016: 4212–4219.
Frey S, et al. Synthesis and Characterization of a Functional Intact IgG in a Prokaryotic Cell-Free Expression System. Biol. Chem. 389(1) 2008: 37–45.
Gregorio NE, et al. Unlocking Applications of Cell-Free Biotechnology Through Enhanced Shelf-Life and Productivity of E. coli Extracts. ACS Synth. Biol. 2020: in press.
Guerra A, von Stosch M, Glassey J. Toward Biotherapeutic Product Real-Time Quality Monitoring. Crit. Rev. Biotechnol. 39(3) 2019: 289–305.
Gurramkonda C, et al. Improving the Recombinant Human Erythropoietin Glycosylation Using Microsome Supplementation in CHO Cell-Free System. Biotechnol. Bioeng. 115(5) 2018: 1253–1264.
Hodgman CE, Jewett MC. Optimized Extract Preparation Methods and Reaction Conditions for Improved Yeast Cell-Free Protein Synthesis. Biotechnol. Bioeng. 110(10) 2013: 2643–2654.
Jérôme V, et al. Comparison of Cell-Based Versus Cell-Free Mammalian Systems for the Production of a Recombinant Human Bone Morphogenic Growth Factor. Eng. Life Sci. 17(10) 2017: 1097–1107.
Kai L, et al. Artificial Environments for the Co-Translational Stabilization of Cell-Free Expressed Proteins. PLoS One 8(2) 2013: e56637.
Kai L, et al. Preparative Scale Production of Functional Mouse Aquaporin 4 Using Different Cell-Free Expression Modes. PLoS One 5(9) 2010: e12972.
Kanai T, et al. Cell-Free Protein Synthesis at High Temperature Using a Lysate of a Hyperthermophile. J. Biotechnol. 126(2) 2006: 185–195.
Kawasaki T, et al. Efficient Synthesis of a Disulfide-Containing Protein Through a Batch Cell-Free System from Wheat Germ. Eur. J. Biochem. 270(23) 2003: 4780–4786.
Kobayashi T, et al. An Improved Cell-Free System for Picornavirus Synthesis. J. Virol. Meth. 142(1–2) 2007: 182–188.
Merk H, et al. Biosynthesis of Membrane Dependent Proteins in Insect Cell Lysates: Identification of Limiting Parameters for Folding and Processing. Biol. Chem. 396(9–10) 2015: 1097–1107.
Merk H, et al. Cell-Free Synthesis of Functional and Endotoxin-Free Antibody Fab Fragments By Translocation into Microsomes. Biotechniques 53(3) 2012: 153–160.
Oh S-J, et al. Translational Incorporation of Multiple Unnatural Amino Acids in a Cell-Free Protein Synthesis System. Biotechnol. Bioproc. Eng. 19(3) 2014: 426–432.
Schoborg JA, et al. Substrate Replenishment and Byproduct Removal Improve Yeast Cell-Free Protein Synthesis. Biotechnol. J. 9(5) 2014: 630–640.
Schoborg JA, et al. Yeast Knockout Library Allows for Efficient Testing of Genomic Mutations for Cell-Free Protein Synthesis. Synth. Syst. Biotechnol. 1(1) 2016: 2–6.
Shin J, Jardine P, Noireaux V. Genome Replication, Synthesis, and Assembly of the Bacteriophage T7 in a Single Cell-Free Reaction. ACS Synth. Biol. 1(9) 2012: 408–413.
Stafford RL, et al. In Vitro Fab Display: A Cell-Free System for IgG Discovery. Prot. Eng. Des. Sel. 27(4) 2014: 97–109.
Stech M, et al. A Continuous-Exchange Cell-Free Protein Synthesis System Based on Extracts from Cultured Insect Cells. PLoS One 9(5) 2014: e96635.
Stech M, et al. Cell-Free Synthesis of Functional Antibodies Using a Coupled In Vitro Transcription-Translation System Based on CHO Cell Lysates. Sci. Rep. 7(1) 2017: 12030.
Stech M, Kubick S. Cell-Free Synthesis Meets Antibody Production: A Review. Antibodies 4(1) 2015: 12–33.
Underwood KA, Swartz JR, Puglisi JD. Quantitative Polysome Analysis Identifies Limitations in Bacterial Cell-Free Protein Synthesis. Biotechnol. Bioeng. 91(4) 2005: 425–435.
Vilkhovoy M, et al. Sequence Specific Modeling of E. coli Cell-Free Protein Synthesis. ACS Synth. Biol. 7(8) 2018: 1844–1857.
Yin G, et al. Aglycosylated Antibodies and Antibody Fragments Produced in a Scalable In Vitro Transcription-Translation System. mAbs 4(2) 2012: 217–225.
Corresponding author Beatrice Melinek, Noelle Colant, Christos Stamatis, Suzanne S. Farid, and Daniel G. Bracewell are in the department of biochemical engineering at University College London (UCL), London WC1E 6BT, UK; b.melinek@ucl.ac.uk. Christopher Lennon is a subject matter expert in molecular and microbiology with Fujifilm Diosynth Biotechnologies, Belasis Avenue, Billingham, TS23 1LH, UK. Karen Polizzi is a reader in biotechnology for the department of chemical engineering at Imperial College London, South Kensington Campus, London SW7 2AZ. And Mark Carver is an independent consultant and Chair of UCL’s Future Targeted Healthcare Manufacturing Hub User Steering Committee.