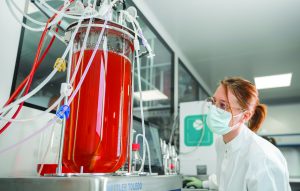
Photo 1: Production of 15 billion human induced pluripotent stem cells (iPSCs) in a 10-L bioreactor using the TreeFrog Therapeutics C-Stem technology, with 276-fold amplification in 6.59 days.
Induced pluripotent stem cells (iPSCs) are promising starting materials for several clinical and industrial applications in cell therapy production, drug discovery, and in vitro screening. But as I learned from Maxime Feyeux (cofounder and chief science officer of TreeFrog Therapeutics in Bordeaux, France), such cells are a relatively new option in the toolbox of cell therapy developers. Advanced-therapy products can require controlled proliferation and differentiation of millions or even billions of iPSCs depending on the clinical indication, he pointed out, yet culture methods and technologies for their stable mass production remain in early development stages (1, 2). Thus, drug developers working with iPSCs today must choose between scaling out production using stacks of two-dimensional (2D) culture vessels or scaling up to three-dimensional (3D) bioreactors.
I spoke with Feyeux in January 2022 to explore considerations for increasing iPSC production. We focused especially on the needs of iPSCs during cell culture processes and the limitations associated with 2D culture systems, which remain the standard in the cell therapy industry. We also discussed how his company’s C-Stem cell-encapsulation technology could promote iPSC expansion and differentiation in 3D culture formats, facilitating scale-up and improving stem-cell quality in turn.
Feyeux was among the first doctoral students in France to work with PSCs and explore their applications in neurology. He performed that research at the Institute for Stem Cell Therapy and Exploration of Monogenic Diseases (i-Stem) in Corbeil-Essonnes, France. While completing his doctoral thesis, he also cowrote a seminal paper on the genomic integrity of PSCs, focusing on selection of the oncogenic 20q11.21 mutation over prolonged culture (3). Feyeux continued his research in the field of PSCs at the University of Geneva in Switzerland, modeling Huntington’s disease genetic dysregulation and investigating PSC-based therapeutics approaches.
The Need for iPSCs
What features make iPSCs especially promising for cell therapy production? Developers of cell therapies need cells that can proliferate and differentiate into a desired phenotype. We also want cells that can be sourced without ethical or regulatory challenges. PSCs are promising because they address those needs. They can proliferate widely enough to start a production process. They can differentiate into any cell type. You can edit PSCs genetically. And although such cells used to be accessed through embryonic sources, now the same type of material can be sourced in ways that are not ethically challenging.
Access to iPSCs is quite recent. The first publication about human PSCs induced from adult somatic cells dates back only to 2006 (4, 5). So iPSCs represent a recent strategy for addressing the age-old problem of cell loss through injury, disease, or aging. We now have the means to understand loss of function in an organ scientifically — for instance, by identifying which cells behave problematically in a given disease. We are getting faster than ever at discovering problems at the cellular level, and we are increasingly capable of generating and studying cells, especially through applications of iPSCs.
Developers of iPSC-based cell therapies definitely stand on the shoulders of giants. Researchers have done important work in immunooncology with
T cells and other starting materials. But having easy access to human PSCs that can differentiate into any cell type enables researchers to think about therapies that are truly “bankable†— off-the-shelf therapies that can reach mass markets and be made immediately available.
Autologous cell therapies take a long time to process, and if a patient needs treatment right now, that person can’t wait three months to reprogram and differentiate cells, then qualify the final product. PSCs enable you to perform an industrialized process. That’s the way to have a true drug product in the cell therapy field. You can develop a reproducible process, your source material can be controlled, and you can manufacture off-the shelf products. You can almost consider autologous cell therapy as a service, whereas iPSC-based therapies are products with consistent quality.
How would you describe current scalability needs for iPSC production? The needs are diverse. Different indications require different amounts of cells and batch sizes. Consider Parkinson’s disease (PD). Per patients in a best-case scenario, you can get away with ~70,000 cells. To treat a diseased liver, you need 5–20% of its weight in cells — at least 10 billion cells. So there can be an amplitude of 10,000- or even 10,000,000-fold between the needs of the products with the lowest and largest numbers of cells. The number of patients affected by PD, though growing, is much lower than for heart disease. Cell therapy developers must have technology that can address such needs — something that can perform from research to production scales.
Scalability needs also differ by the type of cell that ultimately needs to be produced — e.g., natural killer (NK) and chimeric antigen receptor (CAR) T cells for immunooncology. You might need to produce hundreds of millions of such cells. Again, that amount can change a lot depending on indication.
Researchers are now considering how therapeutic cells are delivered. Ideally, all cells should engraft and demonstrate functionality on par with normal cells. Achieving that can be difficult, but if managed, it means much lower doses for the same effect. In the case of immunooncology, a dose could be diminished significantly — e.g., from hundreds of thousands of cells to below the thousand-cell mark.
Currently, developers can produce at most a few billions of cells per batch. But treating heart or liver failure or another “high-titer indication†requires hundreds of billions of cells. And clinically speaking, you must have enough material to treat at the very least 10 patients per batch. Even that does not address the scale. You ideally need 1,000 doses per batch.
Culture Concerns
What special considerations arise during iPSC modification and culture? You don’t want an arm growing on your head or something like that, so when something goes wrong in a PSC’s development, small mistakes are ruled out through apoptosis. Thus, iPSC development is important, and a cell-culture step needs to be very gentle. Such cells need to grow together; they don’t like to be alone. They also dislike being challenged. For example, genetic editing can be difficult because a double-strand break in the genome would be much more impactful in iPSCs than in many other cells. Thus, genetic fine-tuning is much more stringent for iPSCs than it would be for nearly any other cell type. So there are specific concerns.
Most cell therapy developers cultivate PSCs in 2D vessels; some companies produce them in 3D bioreactors as “clusters.†PSCs are passaged serially to reach desired quantities. We prefer to keep the number of passages and accumulations of cell cycles as low as possible because no cell is perfect. That’s a key feature of our C-Stem technology. We can mass-produce iPSCs while controlling the number of passages because almost no cells die in the process.
In the context of development, it makes sense to invest in maintaining genetic integrity through the first few cell doublings. Indeed, PSCs give rise to the whole body, and any mistake will be deeply influential. Hence, pluripotent cells are very good maintaining their genomic integrity, but they need a proper environment to do so. They need a highly protective one, akin to what happens in the body. So developers also need to minimize mechanical stress during culture.
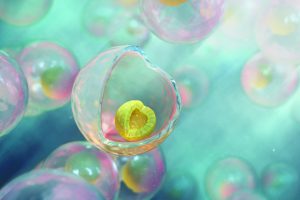
Photo 2: Representation of human iPSCs self-organized in three dimensions within a C-Stem–generated alginate capsule (a proprietary technology of TreeFrog Therapeutics).
How would you describe your company’s C-Stem encapsulation technology? C-Stem–generated capsules are alginate hydrogels that protect iPSCs during a culture process. Each capsule is seeded with a few stem cells, which expand into many cells as a culture progresses (Photos 1 and 2) (6).
Our goal in developing the technology was to give pluripotent cells maximal potential. You need to pamper them to express that potential, reproducing as much as possible the conditions that they experience in vivo. If you give them conditions akin to what they are supposed to find in a human body, then it stands to reason that the cultured cells will do their jobs.
Researchers already know how to grow cells in culture media that resemble the extracellular medium present in a human body. Heating cells to an optimal temperature has been mastered, as has humidity control. And commercially available culture systems perform many of their functions well. But such systems have trouble recapitulating iPSC topology. PSCs are supposed to organize as lumenized epithelium — as a monolayer that folds in on itself. Such an arrangement controls the molecular content of the cluster.
C-Stem capsules are designed not only to protect cells from stresses in their culture environment, but also to help them organize in 3D, as they would do in vivo. We also wanted to create a scale-independent culture system so that small- and large-scale culture processes would yield cells of similar quality. 3D bioreactors can expand cells well, and process engineers can control a reactor’s oxygen levels, humidity, and culture homogeneity — but the most salient culture parameter for iPSCs is shear stress. Protecting cells behind a “firewall,†we thought, could make mechanical stress a moot point. The alginate nature of the capsule is strong enough to protect cells yet porous enough to enable diffusion of gases, culture media, growth factors, and metabolites. So encapsulated iPSCs do not experience shear stress, and they can’t be harmed, say, by hitting an impeller.
Encapsulation also prevents problems with fusion. At large scales, suspension-culture fluids move around quickly, but materials in small-scale cultures do not — and if you don’t keep culture fluids moving enough, cell clusters can fuse.
Stem cells must adhere to a substrate. That can happen in 2D culture vessels, but hard surfaces pose challenges for PSCs. In a C-Stem capsule, iPSCs can proliferate in 3D under conditions that are much closer to the mechanical properties of tissues than they are to plastic. Again, that enables cells to organize into a physiologically relevant lumenized epithelium.
We also can retrieve cultured cells easily. The alginate capsules are reticulated hydrogels that form bonds with calcium. If you remove the calcium, then the gel dissolves back into the culture solution, and you can harvest the cells that grew inside them. C-Stem encapsulation basically enlists physics to protect stem cells and make the best of 3D bioreactors’ advantages for iPSC expansion.
How might C-Stem technology address the scalability needs you described? You certainly need sufficient quantities of iPSCs to treat the condition that you’re trying to deal with. But you also want scalable technologies to ensure that you are not limiting the sizes or numbers of batches that you
can produce.
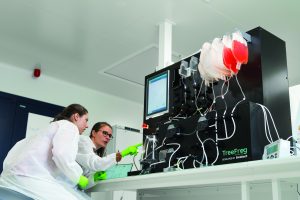
Photo 3: TreeFrog Therapeutics’s industrial device generates over 1,000 stem-cell capsules per second.
The C-Stem encapsulation system can produce thousands of capsules per second (Photo 3). Thus, at the beginning of a culture process, you are working with a few thousand cells per second, but over a few days, those cells amplify hundreds of time. So encapsulation is not the rate-limiting point of the process. A reactor can be seeded with encapsulated cells within an afternoon. Proliferation can take about a week. That’s normal and probably necessary; I would be worried about anything that would speed up proliferation further because cells need time to replicate their DNA properly. We demonstrated in 2021 that a 10-L bioreactor seeded with 50 million stem cells can yield 15 billion cells, which provides enough raw material for 10,000 doses for a PD treatment. That is a significant total. And the encapsulation device works at such high throughput that it is not a limiting factor. Encapsulation actually facilitates cell therapy manufacturing because cells are protected throughout the process.
Why is it important to enable iPSCs to organize into monolayers within their respective capsules? Recapitulating the lumenized growth pattern enables cultured cells to have normal paracrine and autocrine functions, which are important for intercellular signaling. A “monolayer†is not just a layer of cells folded in on itself; all the cells in that layer access the lumen equally. Within a C-Stem–generated capsule, the apical side of each cell accesses the lumen, while the basal side interacts with the surrounding microenvironment.
In 2D culture, cells adhere to plastic. When a cell’s apical side faces the culture medium, its paracrine and autocrine signals dilute away across the whole volume of the media. It’s a bit like shouting to someone across the mountains. Enabling cells to form into a lumenized epithelium, as they would in vivo, ensures that we don’t lose those naturally occurring signals. It is estimated that concentrations of paracrine and autocrine signals are 2,500 times higher in the lumen of stem cell epiblasts than you might find in the supernatant of 2D cultures.
That makes a big difference in terms of iPSC yield and quality. In 2D cultures, dead cells float around, and no one is shocked. Researchers are accustomed to that. But large numbers of dead cells are a clue that something is wrong with your culture. Certainly, cells die naturally, but this does not occur for every division. In a culture with C-Stem capsules, cells proliferate behind a firewall. Dead cells cannot diffuse out. So they remain inside their capsules; they are not rinsed away. But we are finding no dead-cell accumulation. That is critical to what we do. If you are not losing cells along the way, after seven days of cultivation, your cells can amplify at least 100 times the original number. It’s like compound interest: You go from losing part of your cells after each division to losing none, and those savings can go a long way. Each division indeed yields two cells. That’s much better than if you are averaging 50–70× amplification over a week of cultivation, as might happen in a 2D culture system.
That outcome also helps us to manage cell quality. If cells bear a modification that makes them proliferate faster or survive better, but the population at large is dying a lot, then there is significant selection pressure. But if the majority of cells are safe and proliferating happily, then subpopulations with undesired mutations have a much harder time getting an edge over normal cells. Thus, better survival rates mean higher yields and improved product quality.
The Merits of Scale-Up
How do such capabilities play into your company’s scalability strategy? Let’s assume that you are using 2D culture vessels and that your cells are amplifying 70-fold during each culture process. Doing that three times in a row will yield many, many cells that you have to maintain in many, many vessels. Operationally, that workflow might be fine the first time. But to address demand for cell therapy products, you need to deal with the same process at much higher scale between each cell passage, quickly rendering the process unmanageable. Clearly, scaling out can be limiting in terms of productivity.
A company also needs scientists who are skillful at cell culture, and you can’t replicate people. A skilled employee could handle a few square-meters worth of stacked 2D culture equipment. But how are you going to clone your operator to handle more? Some companies answer that question with automation. That is a fair strategy, but it doesn’t solve the issue of nonlinear capacity needs. When scaling out, you might have capital and starting material at the ready, and you might adjust logistics for tiered production. You can develop a lot of strategies, but needing to do that is an issue in and of itself.
We are trying to address such limitations with the C-Stem technology. Working with stirred-tank bioreactors instead of 2D vessels helps us to maximize operator time and cleanroom space. By finding a production method that can scale up easily from a 1-L to a 10-L bioreactor — and soon a 100-L reactor — a therapy developer can begin answering how to deal with the exponential growth that will happen when needing to handle large demands.
Time in culture is another factor to consider when scaling up or out. PSCs need time to proliferate, but you want to optimize that schedule. Fewer divisions mean fewer cell cycles and thus less opportunity for things to go wrong. Each replication of a cell’s genome raises risk for an error. If you can cram an expansion step into one week — which equals seven to nine divisions — then you can harvest your billions of cells instead of growing them over three weeks and introducing at least three times the risk as selection joins the dance and expands de novo mutations. Cell survival is the culture parameter that developers should prioritize. High rates of survival can lead to large yields and high product quality.
Some companies have tried culturing iPSCs in 3D as spheroids, but those don’t promote cell survival. In head-to-head comparisons using standard protocols, we found that spheroid-based cultures in bioreactors actually perform 5,000 times worse than 2D cultures do when amplifying iPSCs over 28 days. That shortfall might come from contact inhibition between cells, or maybe cells inside spheroids have trouble accessing culture media. Other concerns include fusion issues and cell death upon shock events in a bioreactor, and several compounding factors might add to those.
That issue raises a conundrum: Moving production to a suspension-culture bioreactor seems to be the solution to scalability, but it results in a proliferation rate below what would be expected in a 2D environment. If you go 2D, then you have the problems associated with scale-out. So it’s a conundrum. Our answer to the problem is using C-Stem capsules to protect iPSCs, provide them with an in-vivo–like environment, and ease their access to culture media. This solution combines high yield and high scalability in one package.
How does your team control iPSC differentiation? We’ve had success using C-Stem capsules not only to amplify iPSCs, but also to differentiate them. The cell therapy field is turning increasingly toward suspension culture to scale but is having trouble with reproducibility. Control of construct size is key, physically speaking. The diffusion time of differentiation factors differs considerably between constructs of a few microns and a few millimeters. Micrometers in size are okay, hundreds of micrometers start to be clunky, and sizes of millimeters mean that you don’t control anything. We’ve come to master that aspect of the process. We simultaneously protect and control the size of cell constructs, and we can do so irrespective of bioreactor agitation parameters. That gives leeway for chemistry, manufacturing, and controls (CMC) process engineers to fine-tune bioreactor protocols for maximum efficiency using those freed parameters.
What other factors should developers consider relating to product quality and scalability? 2D cultures produce single cells, but human cells do not like to be by themselves in liquid suspension. They might struggle to integrate with a patient’s tissues during transplantation. If that happens, then many of the cells that you’ve worked to produce are going to die. Transplanting microtissues can speed up engraftment because that strategy taps into a normal cellular behavior — a kind of desire to be surrounded by friends. Cells in microtissues also replicate the size (~100 µm) of the building blocks of organs that are resilient in the face of injury and disruption.
Pluripotency is another consideration when scaling up production. Some teams have managed to produce large iPSC batches (>15 billion cells) in large-scale bioreactors. But when you look at the quality attributes of those batches, you find that the cells’ pluripotency plummeted. We have shown with two iPSC cultures (cell line 15b) performed in 10-L bioreactors that pluripotency remains robust when using C-Stem–generated capsules (>96%). The large iPSC batches reported in the literature also needed to be fed a lot of material. So such batches might produce high cell totals, but their expansion rates generally have been poor considering what resources they have needed — and if you want to reach 15 billion cells, it’s better to have to start feeding 50 million cells than to have to feed a billion.
PSC production is both a numbers game and a quality game. Your therapy can’t advance through clinical trials if your product doesn’t have the requisite quality, but the same is true if you don’t produce enough cells. During clinical trials, regulators will be examining the reproducibility of your product across several patients — otherwise, the studies will not be predictive of your drug’s outcomes. If numerous people could be treated with your therapy, then you need to plan accordingly for your clinical trials. In many cases, there is no way around having production capacity that is on par with what we have using C-Stem technology.
References
1 Kropp C, Massai D, Zweigert R. Progress and Challenges in Large-Scale Expansion of Human Pluripotent Stem Cells. Process Biochem. 59(B) 2017: 244–254; https://doi.org/10.1016/j.procbio.2016.09.032.
2 Horiguchi I, Masahiro, K-O. Current Developments in the Stable Production of Human Induced Pluripotent Stem Cells. Engineering 7(2) 2021: 144–152; https://doi.org/10.1016/j.eng.2021.01.001.
3 Lefort N, et al. Human Embryonic Stem Cells Reveal Recurrent Genomic Instability at 20q11.21. Nature Biotechnol. 26, 2008: 1364–1366; https://doi.org/10.1038/nbt.1509.
4 Takahashi K, Yamanaka S. Induction of Pluripotent Stem Cells from Mouse Embryonic and Adult Fibroblast Cultures By Defined Factors. Cell 126(4) 2006: 663–676; https://doi.org/10.1016/j.cell.2006.07.024.
5 Takahashi K, et al. Induction of Pluripotent Stem Cells from Adult Human Fibroblasts By Defined Factors. Cell 131(5) 2007: 861–872; https://doi.org/10.1016/j.cell.2007.11.019.
6 Alessandri K, et al. A 3D-Printed Microfluidic Device for Production of Functionalized Hydrogel Microcapsules for Culture and Differentiation of Human Neuronal Stem Cells (hNSC). Lab on a Chip 9, 2016; https://pubs.rsc.org/en/content/articlelanding/2016/lc/c6lc00133e.
Brian Gazaille is associate editor of BioProcess International, part of Informa Connect, PO Box 70, Dexter, OR 97431; brian.gazaille@informa.com; 1-212-600-3594.