Since the late 1980s, studies have shown that plants can manufacture functional transgenic pharmaceutical compounds. Advantages attributed to plant-made pharmaceutical (PMP) approaches are compelling, and PMP production continues to attract interest from investors and the biopharmaceutical industry (Table 1). Proposed PMP benefits include proven scalability, high production capacity, limited exposure to human or animal pathogens, lower capital expenditures (CapEx), and decreased operating costs. Those putative advantages have proven to be significant business forces driving continued investor support for PMP ventures. PMP production’s lower cost relative to cell culture provides an opportunity to subsidize more research for additional product development.
Table 1: PMPs in clinical trials as of March 2010*
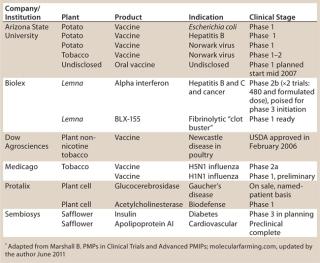
Placed in wider context, the business value, for example, of potentially large production capacity coupled to lower CapEx requirements and manufacturing costs is underscored by concerns over the expanding gap between production volume and patients’ needs for potentially life-saving drugs. In 2010, 178 drug shortages were reported to the US FDA, 132 of which involved sterile, injectable drugs. In 2011, the agency has continued to see an increased number of shortages, especially those involving older sterile injectable drugs (1). So the disparity between production and capacity continues to expand.
Photo 1:
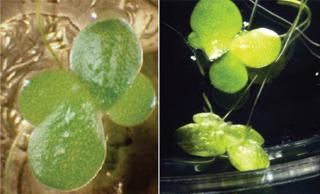
Many situations can create a drug supply shortage, including natural disasters, limited profitability, regulatory activities, and population-based demands. One compelling solution to the growing difference between drug production capacity and patient needs is taking advantage of the simplicity, lowered cost of goods (COGs), increased speed, and greater capacity offered by PMPs.
Other PMP manufacturing advantages are operational and relate, for example, to the risk of animal by-product exposure to cultures, feed streams, and/or active pharmaceutical ingredients (APIs). Manufacturers must demonstrate that their processes and APIs are free of biologically active animal by-products if they are to be approved. For example, prions and viral particles associated with animal-based products may cause serious adverse reactions in humans when injected or ingested. Such exposure can be minimized in well-designed processes using plant-based production cultures and USP Class VI certified components.
With PMP systems, however, the serious concern over animal by-product contamination is reduced or removed completely. They have less inherent animal by-product exposure, thereby eliminating the need for the additional expense of removing associated contaminants. The end result can be simpler, less-expensive processes with more rapid production and development timelines. Historical Perspective of PMP Industry Growth
The possibility of transgenic plant-based pharmaceutical production emerged in the 1980s as advances in genetic engineering reached a critical point and plant genetic transformation became possible. Initial success in transforming plants with genes encoding pharmaceutical compounds engendered a great deal of speculation about the future of PMP manufacturing and its potential benefits to human health, global nutrition, veterinary medicine, and so forth (2).
Since the successful production of the first PMP compound in 1986, plant transformation strategies, upstream processes, and PMP-related regulatory laws have been redefined and improved continually. Although conventional pharmaceutical manufacturing platforms such as yeast and mammalian cell culture continue to yield approved drug products, PMP products have been a rarity. It is not unusual for new technologies to take some time to affect pharmaceutical markets. Despite the acknowledged gradual evolution of the PMP sector, a number of plant-based recombinant drug products are currently poised to move into the final stages of drug approval (Table 1).
Experts have suggested several explanations for the differences between the PMPs’ outlook in the late 1980s and current projections. During the late 1980s and 1990s, PMP opportunities seemed vast and immediate because scientists in industry and academia focused primarily on yield rather than regulatory aspects. Early business models that sprang from this initial excitement proved incomplete. Early PMP researchers envisioned an open, field-based production factory that could be rapidly implemented with simplified scalability, resulting in an equally rapid turnaround in investment capital. That initial vision was gradually brought down to Earth during the following decade because of mounting concerns over genetic containment.
Because of documented incidents of accidental release of or contamination by genetically modified organisms (GMOs, plants in this case), the public has become quite sensitive to the potential for unchecked genetic contamination of the environment. That concern arises from uncertainty regarding what potential damage or catastrophic consequences the release of putative artificial genetic material may cause. Such concerns are legitimate, and manufacturers must verify that proper precautions are in place to contain their GMOs. The necessary containment measures for a given enclosed process are dictated by individual system and facility requirements. Open systems must consider the requirements for proper and sufficient buffer areas — where these requirements have been or can be established. Some requirements for open systems are still in development, making open GMO production systems particularly challenging from a regulatory standpoint.
Photo 2:
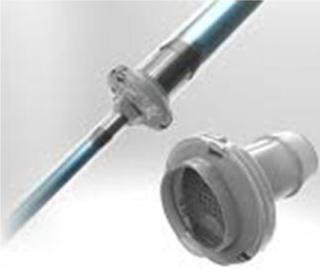
Another reason the PMP sector’s growth has slowed is the US Department of Agriculture’s (USDA) Animal and Plant Health Inspection Services (APHIS) rigorous enforcement of genetic material containment regulations. To protect public interests, regulatory agencies carefully monitor current and future safety concerns associated with GMOs that can produce PMPs and implement appropriate regulations. C
omplying with those legitimately prescribed rules has challenged PMP manufacturing growth.
Regulations designed to protect the public food supply and ecological genetic stability have created industry concerns over the cost and complexity of effective containment strategies (3). Facing progressively tighter environmental regulations, many PMP research operations are investigating the use of greenhouses, enclosed hydroponic-based systems, and other designs in which environmental contamination is minimized to reduce regulatory complexity (4).
Some companies continue to develop crop-based production platforms (e.g., Sembiosys, Medicago, and iBio). Other approaches attempt to adapt platform species to regulatory requirements through enclosed, cell-based liquid culture platforms using modified bioreactors, lighted tanks, and other aspects of conventional cell culture processes (e.g., Protalix). Notably, another class of processes in development suggests that systems familiar to the FDA provide the only feasible route to pharmaceutical manufacturing and regulatory success. Biolex Therapeutics Inc. of North Carolina is actively exploring such technological opportunities.
Photo 3:
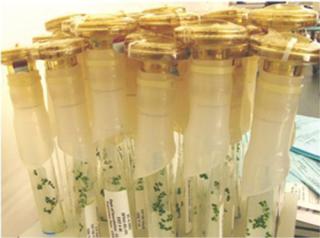
Table 2: Scale has little effect on the LEX System culture growth rate or yield per unit fresh weight. As indicated, scaling up the process gives repeatable biomass-to-yield ratios. Correlations between production volume and yield can falter when typical production systems are scaled. However, in this case, the Lex System technology shows no decline in yied proportional to scale.
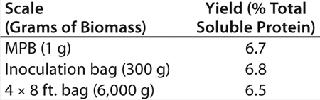
PMPs are typically derived from methods of transgenic protein expression in plants. Among the differing plant platforms are two relatively distinct categories of transformation approaches: stable transgene expression and transient transgene expression. The Biolex stable transgene manufacturing process, termed LEX System (or Lemna Expression System), has been proven through good manufacturing practice (GMP) production runs for now completed and successful Locteron phase 1, 2a, and 2b clinical trials.
Photo 4:
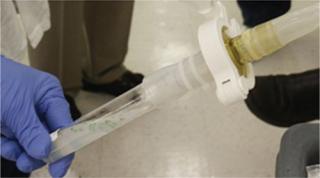
Photo 5:
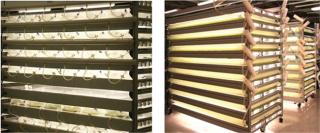
A Novel Approach
Biolex is a privately owned, clinical-stage biopharmaceutical company that uses proprietary technology to develop pharmaceutical proteins, including glycan-engineered monoclonal antibodies, biosimilars, follow-on biologics, and veterinary medicines (5). The LEX System process uses genetically transformed cultures of Lemna minor (duckweed) in manufacture of those products. As a plant-based production system, it addresses the need for control, containment, and cost reduction. The system has produced pharmaceutical compounds proven effective through phase 2 clinical studies. The current drug product made with LEX System technology (developed in conjunction with Netherlands-based Octoplus NV) is Locteron, a controlled-release interferon formulation for treating Hepatitis C (6,7,8).
Having used a relatively common species of aquatic monocot (Photo 1) to create a PMP manufacturing process, Biolex integrated its process into a single-use systems (SUS) approach. Adding SUS materials to the original PMP approach further reduced compliance concerns by nearly eliminating any need for cleaning validation upstream. Leveraging Single-Use Products: Benefits and Compliance
Combining PMP production processes with a new generation of single-use products presented an opportunity to the disposables industry. The synergies of those technologies became all the more compelling as requirements for plant genetic containment increased their hold on the PMP industry. In 2004, Biolex began testing bags for use as manufacturing culture vessels, biomass sequestering and filtration systems, and manifolds.
Each stage of the production process is designed to be closed and aseptic to build on the already animal-product-contact-free aspect of plant-based cultures. To further reduce cost and complexity, the design ensures that no classified areas are required for the upstream process. LEX System technology is designed to prevent contamination from all microorganisms and biocontaminants through the application of disposable aseptic connectors and gamma-sterilized single-use seed bags, production bags, harvest bags, and tubing assemblies. In addition, harvest has been simplified through application of a proprietary single-use design to create a fully disposable, low-cost material separation and sequestration step. With a tested and proven aseptic process, this technology stands exemplifies pharmaceutical process containment and control.
The single-use process addresses animal-product-free requirements, including extractables and leachables control through strict use of USP Class VI materials in the process stream. Using Class VI disposables hands off to SUS vendors a large part of the compliance, documentation, and regulatory control burden. Additional regulatory support is handled by in-house and external material and product testing, vendor auditing, and document management. All FDA and EMEA regulations pertaining to SU
S are strictly followed, and compliance control is built into the upstream process through a rigorous documentation and SOP management system.
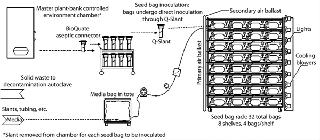
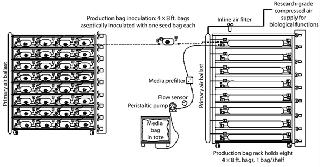
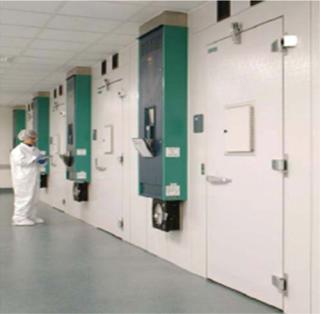
One of two distinct advantages of this production approach is that it transfers part of the disposables quality responsibility to vendors. The second advantage is that consistent application of single-use products throughout upstream processes eliminates all cleaning validation requirements for process stream hardware. That is a significant cost savings for Biolex and goes far toward offsetting the cost of disposables-based operations.
Regulatory Guidelines
Single-use regulatory guidelines cover bag materials in general and specific product-contact layers (11).
All Single-Use, GMP Materials
USP Class VI Cytotoxicity and Physicochemical Standards; USP Class VI Material Certificate, signed and dated (S&D).
Documentation with Certificate of Origin of Materials provided for all product contact materials; (S&D)
TSE, BSE free — for example, a statement should be provided that bovine-derived materials from bovine spongiform encephalopathy (BSE) countries or any products exposed to transmissible spongiform encephalopathy (TSE) agents (as defined by the US Department of Agriculture, 9 CFR 94.11) are not used or manipulated in the same facility as any of the materials of construction for the bag assembly (S&D)
All materials of construction must be animal-derived-component free (ADCF), (S&D)
Extractable and leachable screening results and documentation must be available as needed for pre- and poststerilization testing
Materials are gamma stable
Materials are UV stable
Additional Requirements for Product-Contact Layers
In addition to the above requirements, the following requirements are also applicable for bag product-contact layers:
Hemolysis ISO 1993-4 2002
Physicochemical USP28
Cytotoxicity MEM Elution, USP 28
Bacterial endotoxin; LAL <0.25 EU/mL extraction media, pass
Moisture permeability, E-96-90 method, <0.3 g/100 in 24 hours The LEX System Process
Lemna (commonly known as duckweed) is an aquatic monocotyledonous plant that thrives in the top 1 cm of still, freshwater systems. Several of its characteristics make it ideally suited for PMP production (4), including its
-
ability for clonal reproduction
-
high protein content
-
rapid growth rate
-
classification as a nonindustrialized plant, not a food crop
-
growth in a wide range of conditions
-
ability to produce secreted or tissue-bound products.
-
In addition, Lemna‘s process advantages include
-
viral inactivation steps are not required
-
scale has no effect on yield per unit weight or protein quality
-
no pesticides, herbicides, or antibiotics are used in production cultures.
Clonal reproduction simplifies line management, propagation, and the process feed stream. The absence of pollen or flowers simplifies genetic containment. Lemna‘s high total soluble protein (TSP) content provides higher expression and target protein concentrations per unit biomass than other PMP platforms (9). Another strategically advantageous characteristic is its culture growth rate. Lemna doubles its biomass every 36 hours under normal growth conditions, a doubling rate that exceeds other plant systems biomass build rates. Process Control
Photosynthesis converts sunlight to energy for cellular processes, in which carbon dioxide is converted to biomass and cellular products such as target transgenic proteins. Consequently, to exploit Lemna‘s growth rate and high levels of expression, cultures must receive appropriate amounts of light and filtered ambient air. Other important environmental parameters are media composition and temperature.
In processes, facilities, and hardware designs, only those four critical environmental factors are considered. This simplicity goes far toward limiting overall process and hardware design complexity and final facility costs. The LEX System upstream process is in definite contrast with complex photobioreactor systems that require monitoring of many physical indices, precision controls, and cleaning validation.
Design of experiment (DoE) software established critical ranges for environmental parameters. Studies tested ranges for yield, repeatability, and robustness. A study of failure mode and effects analysis (FMEA) used a scaled-down model system. That model addressed the range of possible failures, effects of each failure on product quality and yield, and critical points for process failure responses. Those process ranges and failure data provided process design standards and established system robustness. A scaled-up manufacturing system confirmed those results.
In addition to environmental testing, DoE addressing media composition, storage life, and postharvest FMEA added further critical guidelines for media preparation and vessel filling processes. Criteria for product storage were developed through HPLC quality analysis of storage regimes for harvested media and tissue. Those data were added to the overall process design to set hold-step requirements and facilitate raw-product transfer method controls.
Intensive collaboration among upstream process development, quality assurance (QA), and regulatory and operations functions throughout development of the production format was necessary to ensure minimized requirements for redesigns. This ensured careful considerations of regulations from the FDA and European agencies at all stages of development. For example, the potential for animal-product exposure was designed out of the process, as were any activities difficult to validate or hard to control and document. Lot definition requirements also played an important role in developing
the best and most compliant lot characterization.
Regulators have responded to this single-use, plant-based process with several comments and observations (10):
-
simplicity, few critical process parameters (CPP)
-
no animal-derived components in the process or media
-
no viral inactivation or prion clearance measures required
-
closed system: upstream processing can be in unclassified area
-
most special GMP guidances for transgenic plants do not apply
-
no open field production
-
no herbicides, pesticides, antibiotics, or environmental bioburden
-
no pollen or seeds due to clonal propagation.
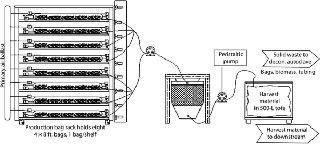
Single-Use Component Benefits and Requirements
This plant-based process uses proprietary culture bags designed to meet vertical and horizontal stacking density and scaling requirements. All filters, aseptic connectors, ports, and pressurized systems undergo extensive in-housing testing for baseline characteristics, flow volume and rate, burst pressure, barrier effectiveness, and product consistency.
The “Regulatory Guidelines” box provides an example of regulatory materials of construction (MoC) requirements for the disposable, production-scale vessels.As indicated in that list, animal-contact–free MoC are of primary importance. All products are certified animal-product–free before any purchases for GMP operations. Each approved item is shipped from its vendor with a certificate of authenticity (C of A), Certificate of conformity (C of C), lot number, and sterilization records.
One important component that helped Biolex design its enclosed, aseptic upstream process was the latest generation of aseptic connectors (Photo 2). When properly used, these connectors (BioQuate ReadyMate) are reported to provide an aseptic/sterile connection while requiring no classified areas or external sterile air-flow system.
Based on that design, process connections between bags, for example, could be made simply. Aseptic integrity of the components could be ensured despite gray-space operation. This type of aseptic connector proved a critical factor in lowering facility costs and simplifying process and hardware design. Due to their strategic role — and to prove compliance and control — it was necessary for those connectors to undergo a comprehensive testing regimen that included bacterial challenges, consistency testing, durability analysis, and additional FMEA testing. Process Flow
Upstream production begins with a master plant bank (MPB), the plant analogue of the more commonly known “master cell bank” (Photo 3). The LEX System process has demonstrated that small numbers of fronds can be stored in agar slants at 8–10 °C for up to a year with full viability and no genetic drift. To further control and preserve the plant bank, LEX System technology relies on a proprietary cryopreservation-based living line archival system. Although conventional archive systems have proven plant viability through nine years, cryopreservation systems have the potential to store recoverable plant material for much longer, possibly 20 years or more.
This upstream process begins when the contents of slants are transferred to “seed bags” (Photo 4, Figure 1). Once inoculated and supplied with media, the seed bags are placed on a lighted rack and attached to a compressed air supply. Other than temperature control through rack design and production-suite air handling, the only environmental support these cultures require are compressed air for biological processes and light for photosynthesis. The support rack is a proprietary, customized, self-contained lighting system with bag restraint, temperature control, and air distribution built in (Photo 5). Each rack can provide temperature feedback to the HVAC system for complete homeostatic control.
When the seed bag incubation cycle is nearly complete, in-process testing (IPT) samples are used to detail the integrity of the cultures. All bags with bioburden are removed from the process stream. Once seed-bag incubation is complete, those bags are connected to production bags through aseptic connectors (Figure 2). The contents of the seed bag are decanted into the production bag, and the connection between the two is sealed and cut (Figure 2). Used seed bags are deactivated through sterilization and processed for waste or materials recycling.
As production cultures reach maturity, samples are removed through the sample port and sent to QC for analysis. Bioburden-free bags continue in the process stream to harvest. Because the process is robust, contamination is very rare.
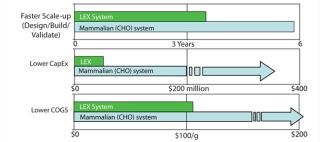
During harvest of the production bags, pumps move media and tissue through a proprietary, disposable “harvest bag” (Figure 3). While the harvest bag captures and encloses solid materials, media flow through the bag’s filtration panel and are pumped to a large collection tote for transfer to downstream processing. In a sealed single-use tubing network, the content of each bag is protected from contamination throughout the harvest process (as with the rest of the upstream process). If target proteins are secreted into the media, fronds sequestered in the harvest bag are decontaminated and discarded per USDA regulations. This production process requires none of the stirring or active control of media constituents, pH, or CO2 tension that many conventional batch processes require.
Once recovered, media are removed and sent downstream for clarification and additional purification into API. If a target protein is tissue bound, then harvest bags containing biomass and product are transferred to downstream processing. That involves simple disruption to extract protein from the biomass. Often a low-pH precipitation can significantly reduce host proteins and remove phenolic compounds. Depending on scale, either centrifugation or depth filtration clarifies the extract. Standard, relatively low-cost equipment is used for those steps, and no viral clearance is required. The rest of the downstream process is typical and depends on the target protein. For example, protein A capture is used for antibodies followed by one or two additional chromatography steps. Either biomass or media can be frozen and stored for later processing, if desired. Effective PMP System
LEX System technology offers several advantages over other systems (Figure 4). It minimizes the risk of accidental genetic release of product or contamination of food sources. Although nontoxic and edible, Lemna is not commonly used as a food source. A further advantage is that no pesticides, herbicides, or antibiotics are used in Lemna cultu
res. In addition, the single-use aspect of this production system has also nearly eliminated cleaning validation activities from upstream processes.
The current single-use process has handed off materials and product validation responsibility to vendors and manufacturers of disposable products. This has provided additional benefits of reduced work-force requirements and documentation burden.
Using disposable aseptic connectors eliminates the need for classified areas in upstream processes. This reduces facility and operating costs and process complexity. Aseptic connectors provided for a process that can specify and achieve low contamination levels, well within process requirements. In receiving a contaminant-free feed stream, downstream processing benefits as well.
LEX System technology has merged plant-based pharmaceutical protein production with single-use materials. The benefits and advantages to this approach have been demonstrated through investor support and FDA acknowledgement of successful clinical trials. A crucial synergy has manifested itself in the careful adaptation of single-use products to the Lemna-based PMP technology.
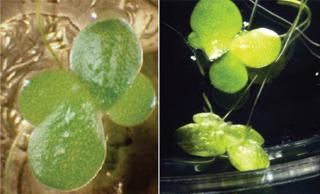
Many situations can create a drug supply shortage, including natural disasters, limited profitability, regulatory activities, and population-based demands. One compelling solution to the growing difference between drug production capacity and patient needs is taking advantage of the simplicity, lowered cost of goods (COGs), increased speed, and greater capacity offered by PMPs.
Other PMP manufacturing advantages are operational and relate, for example, to the risk of animal by-product exposure to cultures, feed streams, and/or active pharmaceutical ingredients (APIs). Manufacturers must demonstrate that their processes and APIs are free of biologically active animal by-products if they are to be approved. For example, prions and viral particles associated with animal-based products may cause serious adverse reactions in humans when injected or ingested. Such exposure can be minimized in well-designed processes using plant-based production cultures and USP Class VI certified components.
With PMP systems, however, the serious concern over animal by-product contamination is reduced or removed completely. They have less inherent animal by-product exposure, thereby eliminating the need for the additional expense of removing associated contaminants. The end result can be simpler, less-expensive processes with more rapid production and development timelines. Historical Perspective of PMP Industry Growth
The possibility of transgenic plant-based pharmaceutical production emerged in the 1980s as advances in genetic engineering reached a critical point and plant genetic transformation became possible. Initial success in transforming plants with genes encoding pharmaceutical compounds engendered a great deal of speculation about the future of PMP manufacturing and its potential benefits to human health, global nutrition, veterinary medicine, and so forth (2).
Since the successful production of the first PMP compound in 1986, plant transformation strategies, upstream processes, and PMP-related regulatory laws have been redefined and improved continually. Although conventional pharmaceutical manufacturing platforms such as yeast and mammalian cell culture continue to yield approved drug products, PMP products have been a rarity. It is not unusual for new technologies to take some time to affect pharmaceutical markets. Despite the acknowledged gradual evolution of the PMP sector, a number of plant-based recombinant drug products are currently poised to move into the final stages of drug approval (Table 1).
Experts have suggested several explanations for the differences between the PMPs’ outlook in the late 1980s and current projections. During the late 1980s and 1990s, PMP opportunities seemed vast and immediate because scientists in industry and academia focused primarily on yield rather than regulatory aspects. Early business models that sprang from this initial excitement proved incomplete. Early PMP researchers envisioned an open, field-based production factory that could be rapidly implemented with simplified scalability, resulting in an equally rapid turnaround in investment capital. That initial vision was gradually brought down to Earth during the following decade because of mounting concerns over genetic containment.
Because of documented incidents of accidental release of or contamination by genetically modified organisms (GMOs, plants in this case), the public has become quite sensitive to the potential for unchecked genetic contamination of the environment. That concern arises from uncertainty regarding what potential damage or catastrophic consequences the release of putative artificial genetic material may cause. Such concerns are legitimate, and manufacturers must verify that proper precautions are in place to contain their GMOs. The necessary containment measures for a given enclosed process are dictated by individual system and facility requirements. Open systems must consider the requirements for proper and sufficient buffer areas — where these requirements have been or can be established. Some requirements for open systems are still in development, making open GMO production systems particularly challenging from a regulatory standpoint.
Photo 2:
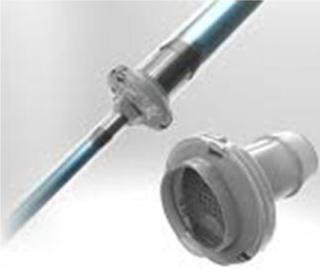
Another reason the PMP sector’s growth has slowed is the US Department of Agriculture’s (USDA) Animal and Plant Health Inspection Services (APHIS) rigorous enforcement of genetic material containment regulations. To protect public interests, regulatory agencies carefully monitor current and future safety concerns associated with GMOs that can produce PMPs and implement appropriate regulations. C
omplying with those legitimately prescribed rules has challenged PMP manufacturing growth.
Regulations designed to protect the public food supply and ecological genetic stability have created industry concerns over the cost and complexity of effective containment strategies (3). Facing progressively tighter environmental regulations, many PMP research operations are investigating the use of greenhouses, enclosed hydroponic-based systems, and other designs in which environmental contamination is minimized to reduce regulatory complexity (4).
Some companies continue to develop crop-based production platforms (e.g., Sembiosys, Medicago, and iBio). Other approaches attempt to adapt platform species to regulatory requirements through enclosed, cell-based liquid culture platforms using modified bioreactors, lighted tanks, and other aspects of conventional cell culture processes (e.g., Protalix). Notably, another class of processes in development suggests that systems familiar to the FDA provide the only feasible route to pharmaceutical manufacturing and regulatory success. Biolex Therapeutics Inc. of North Carolina is actively exploring such technological opportunities.
Photo 3:
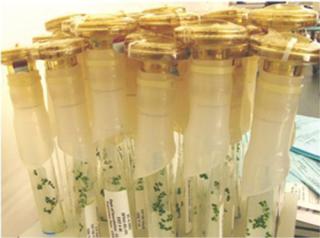
Table 2: Scale has little effect on the LEX System culture growth rate or yield per unit fresh weight. As indicated, scaling up the process gives repeatable biomass-to-yield ratios. Correlations between production volume and yield can falter when typical production systems are scaled. However, in this case, the Lex System technology shows no decline in yied proportional to scale.
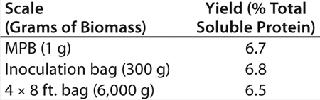
PMPs are typically derived from methods of transgenic protein expression in plants. Among the differing plant platforms are two relatively distinct categories of transformation approaches: stable transgene expression and transient transgene expression. The Biolex stable transgene manufacturing process, termed LEX System (or Lemna Expression System), has been proven through good manufacturing practice (GMP) production runs for now completed and successful Locteron phase 1, 2a, and 2b clinical trials.
Photo 4:
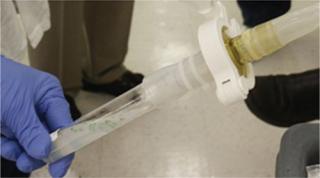
Photo 5:
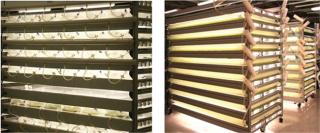
A Novel Approach
Biolex is a privately owned, clinical-stage biopharmaceutical company that uses proprietary technology to develop pharmaceutical proteins, including glycan-engineered monoclonal antibodies, biosimilars, follow-on biologics, and veterinary medicines (5). The LEX System process uses genetically transformed cultures of Lemna minor (duckweed) in manufacture of those products. As a plant-based production system, it addresses the need for control, containment, and cost reduction. The system has produced pharmaceutical compounds proven effective through phase 2 clinical studies. The current drug product made with LEX System technology (developed in conjunction with Netherlands-based Octoplus NV) is Locteron, a controlled-release interferon formulation for treating Hepatitis C (6,7,8).
Having used a relatively common species of aquatic monocot (Photo 1) to create a PMP manufacturing process, Biolex integrated its process into a single-use systems (SUS) approach. Adding SUS materials to the original PMP approach further reduced compliance concerns by nearly eliminating any need for cleaning validation upstream. Leveraging Single-Use Products: Benefits and Compliance
Combining PMP production processes with a new generation of single-use products presented an opportunity to the disposables industry. The synergies of those technologies became all the more compelling as requirements for plant genetic containment increased their hold on the PMP industry. In 2004, Biolex began testing bags for use as manufacturing culture vessels, biomass sequestering and filtration systems, and manifolds.
Each stage of the production process is designed to be closed and aseptic to build on the already animal-product-contact-free aspect of plant-based cultures. To further reduce cost and complexity, the design ensures that no classified areas are required for the upstream process. LEX System technology is designed to prevent contamination from all microorganisms and biocontaminants through the application of disposable aseptic connectors and gamma-sterilized single-use seed bags, production bags, harvest bags, and tubing assemblies. In addition, harvest has been simplified through application of a proprietary single-use design to create a fully disposable, low-cost material separation and sequestration step. With a tested and proven aseptic process, this technology stands exemplifies pharmaceutical process containment and control.
The single-use process addresses animal-product-free requirements, including extractables and leachables control through strict use of USP Class VI materials in the process stream. Using Class VI disposables hands off to SUS vendors a large part of the compliance, documentation, and regulatory control burden. Additional regulatory support is handled by in-house and external material and product testing, vendor auditing, and document management. All FDA and EMEA regulations pertaining to SU
S are strictly followed, and compliance control is built into the upstream process through a rigorous documentation and SOP management system.
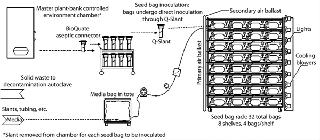
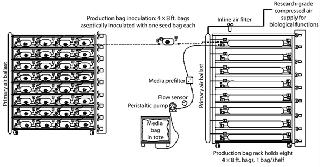
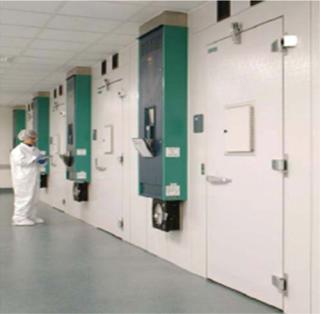
One of two distinct advantages of this production approach is that it transfers part of the disposables quality responsibility to vendors. The second advantage is that consistent application of single-use products throughout upstream processes eliminates all cleaning validation requirements for process stream hardware. That is a significant cost savings for Biolex and goes far toward offsetting the cost of disposables-based operations.
Regulatory Guidelines
Single-use regulatory guidelines cover bag materials in general and specific product-contact layers (11).
All Single-Use, GMP Materials
USP Class VI Cytotoxicity and Physicochemical Standards; USP Class VI Material Certificate, signed and dated (S&D).
Documentation with Certificate of Origin of Materials provided for all product contact materials; (S&D)
TSE, BSE free — for example, a statement should be provided that bovine-derived materials from bovine spongiform encephalopathy (BSE) countries or any products exposed to transmissible spongiform encephalopathy (TSE) agents (as defined by the US Department of Agriculture, 9 CFR 94.11) are not used or manipulated in the same facility as any of the materials of construction for the bag assembly (S&D)
All materials of construction must be animal-derived-component free (ADCF), (S&D)
Extractable and leachable screening results and documentation must be available as needed for pre- and poststerilization testing
Materials are gamma stable
Materials are UV stable
Additional Requirements for Product-Contact Layers
In addition to the above requirements, the following requirements are also applicable for bag product-contact layers:
Hemolysis ISO 1993-4 2002
Physicochemical USP28
Cytotoxicity MEM Elution, USP 28
Bacterial endotoxin; LAL <0.25 EU/mL extraction media, pass
Moisture permeability, E-96-90 method, <0.3 g/100 in 24 hours The LEX System Process
Lemna (commonly known as duckweed) is an aquatic monocotyledonous plant that thrives in the top 1 cm of still, freshwater systems. Several of its characteristics make it ideally suited for PMP production (4), including its
-
ability for clonal reproduction
-
high protein content
-
rapid growth rate
-
classification as a nonindustrialized plant, not a food crop
-
growth in a wide range of conditions
-
ability to produce secreted or tissue-bound products.
-
In addition, Lemna‘s process advantages include
-
viral inactivation steps are not required
-
scale has no effect on yield per unit weight or protein quality
-
no pesticides, herbicides, or antibiotics are used in production cultures.
Clonal reproduction simplifies line management, propagation, and the process feed stream. The absence of pollen or flowers simplifies genetic containment. Lemna‘s high total soluble protein (TSP) content provides higher expression and target protein concentrations per unit biomass than other PMP platforms (9). Another strategically advantageous characteristic is its culture growth rate. Lemna doubles its biomass every 36 hours under normal growth conditions, a doubling rate that exceeds other plant systems biomass build rates. Process Control
Photosynthesis converts sunlight to energy for cellular processes, in which carbon dioxide is converted to biomass and cellular products such as target transgenic proteins. Consequently, to exploit Lemna‘s growth rate and high levels of expression, cultures must receive appropriate amounts of light and filtered ambient air. Other important environmental parameters are media composition and temperature.
In processes, facilities, and hardware designs, only those four critical environmental factors are considered. This simplicity goes far toward limiting overall process and hardware design complexity and final facility costs. The LEX System upstream process is in definite contrast with complex photobioreactor systems that require monitoring of many physical indices, precision controls, and cleaning validation.
Design of experiment (DoE) software established critical ranges for environmental parameters. Studies tested ranges for yield, repeatability, and robustness. A study of failure mode and effects analysis (FMEA) used a scaled-down model system. That model addressed the range of possible failures, effects of each failure on product quality and yield, and critical points for process failure responses. Those process ranges and failure data provided process design standards and established system robustness. A scaled-up manufacturing system confirmed those results.
In addition to environmental testing, DoE addressing media composition, storage life, and postharvest FMEA added further critical guidelines for media preparation and vessel filling processes. Criteria for product storage were developed through HPLC quality analysis of storage regimes for harvested media and tissue. Those data were added to the overall process design to set hold-step requirements and facilitate raw-product transfer method controls.
Intensive collaboration among upstream process development, quality assurance (QA), and regulatory and operations functions throughout development of the production format was necessary to ensure minimized requirements for redesigns. This ensured careful considerations of regulations from the FDA and European agencies at all stages of development. For example, the potential for animal-product exposure was designed out of the process, as were any activities difficult to validate or hard to control and document. Lot definition requirements also played an important role in developing
the best and most compliant lot characterization.
Regulators have responded to this single-use, plant-based process with several comments and observations (10):
-
simplicity, few critical process parameters (CPP)
-
no animal-derived components in the process or media
-
no viral inactivation or prion clearance measures required
-
closed system: upstream processing can be in unclassified area
-
most special GMP guidances for transgenic plants do not apply
-
no open field production
-
no herbicides, pesticides, antibiotics, or environmental bioburden
-
no pollen or seeds due to clonal propagation.
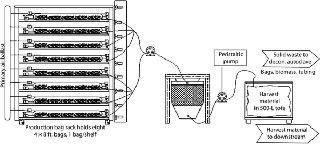
Single-Use Component Benefits and Requirements
This plant-based process uses proprietary culture bags designed to meet vertical and horizontal stacking density and scaling requirements. All filters, aseptic connectors, ports, and pressurized systems undergo extensive in-housing testing for baseline characteristics, flow volume and rate, burst pressure, barrier effectiveness, and product consistency.
The “Regulatory Guidelines” box provides an example of regulatory materials of construction (MoC) requirements for the disposable, production-scale vessels.As indicated in that list, animal-contact–free MoC are of primary importance. All products are certified animal-product–free before any purchases for GMP operations. Each approved item is shipped from its vendor with a certificate of authenticity (C of A), Certificate of conformity (C of C), lot number, and sterilization records.
One important component that helped Biolex design its enclosed, aseptic upstream process was the latest generation of aseptic connectors (Photo 2). When properly used, these connectors (BioQuate ReadyMate) are reported to provide an aseptic/sterile connection while requiring no classified areas or external sterile air-flow system.
Based on that design, process connections between bags, for example, could be made simply. Aseptic integrity of the components could be ensured despite gray-space operation. This type of aseptic connector proved a critical factor in lowering facility costs and simplifying process and hardware design. Due to their strategic role — and to prove compliance and control — it was necessary for those connectors to undergo a comprehensive testing regimen that included bacterial challenges, consistency testing, durability analysis, and additional FMEA testing. Process Flow
Upstream production begins with a master plant bank (MPB), the plant analogue of the more commonly known “master cell bank” (Photo 3). The LEX System process has demonstrated that small numbers of fronds can be stored in agar slants at 8–10 °C for up to a year with full viability and no genetic drift. To further control and preserve the plant bank, LEX System technology relies on a proprietary cryopreservation-based living line archival system. Although conventional archive systems have proven plant viability through nine years, cryopreservation systems have the potential to store recoverable plant material for much longer, possibly 20 years or more.
This upstream process begins when the contents of slants are transferred to “seed bags” (Photo 4, Figure 1). Once inoculated and supplied with media, the seed bags are placed on a lighted rack and attached to a compressed air supply. Other than temperature control through rack design and production-suite air handling, the only environmental support these cultures require are compressed air for biological processes and light for photosynthesis. The support rack is a proprietary, customized, self-contained lighting system with bag restraint, temperature control, and air distribution built in (Photo 5). Each rack can provide temperature feedback to the HVAC system for complete homeostatic control.
When the seed bag incubation cycle is nearly complete, in-process testing (IPT) samples are used to detail the integrity of the cultures. All bags with bioburden are removed from the process stream. Once seed-bag incubation is complete, those bags are connected to production bags through aseptic connectors (Figure 2). The contents of the seed bag are decanted into the production bag, and the connection between the two is sealed and cut (Figure 2). Used seed bags are deactivated through sterilization and processed for waste or materials recycling.
As production cultures reach maturity, samples are removed through the sample port and sent to QC for analysis. Bioburden-free bags continue in the process stream to harvest. Because the process is robust, contamination is very rare.
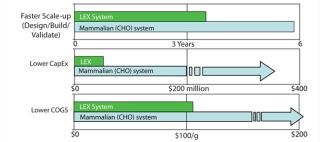
During harvest of the production bags, pumps move media and tissue through a proprietary, disposable “harvest bag” (Figure 3). While the harvest bag captures and encloses solid materials, media flow through the bag’s filtration panel and are pumped to a large collection tote for transfer to downstream processing. In a sealed single-use tubing network, the content of each bag is protected from contamination throughout the harvest process (as with the rest of the upstream process). If target proteins are secreted into the media, fronds sequestered in the harvest bag are decontaminated and discarded per USDA regulations. This production process requires none of the stirring or active control of media constituents, pH, or CO2 tension that many conventional batch processes require.
Once recovered, media are removed and sent downstream for clarification and additional purification into API. If a target protein is tissue bound, then harvest bags containing biomass and product are transferred to downstream processing. That involves simple disruption to extract protein from the biomass. Often a low-pH precipitation can significantly reduce host proteins and remove phenolic compounds. Depending on scale, either centrifugation or depth filtration clarifies the extract. Standard, relatively low-cost equipment is used for those steps, and no viral clearance is required. The rest of the downstream process is typical and depends on the target protein. For example, protein A capture is used for antibodies followed by one or two additional chromatography steps. Either biomass or media can be frozen and stored for later processing, if desired. Effective PMP System
LEX System technology offers several advantages over other systems (Figure 4). It minimizes the risk of accidental genetic release of product or contamination of food sources. Although nontoxic and edible, Lemna is not commonly used as a food source. A further advantage is that no pesticides, herbicides, or antibiotics are used in Lemna cultu
res. In addition, the single-use aspect of this production system has also nearly eliminated cleaning validation activities from upstream processes.
The current single-use process has handed off materials and product validation responsibility to vendors and manufacturers of disposable products. This has provided additional benefits of reduced work-force requirements and documentation burden.
Using disposable aseptic connectors eliminates the need for classified areas in upstream processes. This reduces facility and operating costs and process complexity. Aseptic connectors provided for a process that can specify and achieve low contamination levels, well within process requirements. In receiving a contaminant-free feed stream, downstream processing benefits as well.
LEX System technology has merged plant-based pharmaceutical protein production with single-use materials. The benefits and advantages to this approach have been demonstrated through investor support and FDA acknowledgement of successful clinical trials. A crucial synergy has manifested itself in the careful adaptation of single-use products to the Lemna-based PMP technology.
omplying with those legitimately prescribed rules has challenged PMP manufacturing growth.
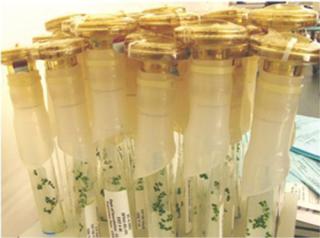
Table 2: Scale has little effect on the LEX System culture growth rate or yield per unit fresh weight. As indicated, scaling up the process gives repeatable biomass-to-yield ratios. Correlations between production volume and yield can falter when typical production systems are scaled. However, in this case, the Lex System technology shows no decline in yied proportional to scale.
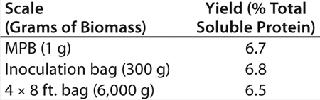
PMPs are typically derived from methods of transgenic protein expression in plants. Among the differing plant platforms are two relatively distinct categories of transformation approaches: stable transgene expression and transient transgene expression. The Biolex stable transgene manufacturing process, termed LEX System (or Lemna Expression System), has been proven through good manufacturing practice (GMP) production runs for now completed and successful Locteron phase 1, 2a, and 2b clinical trials.
Photo 4:
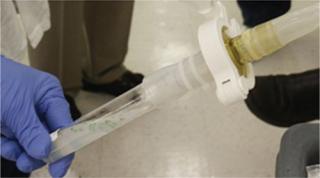
Photo 5:
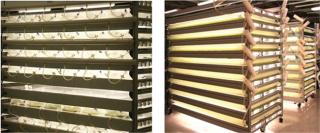
A Novel Approach
Biolex is a privately owned, clinical-stage biopharmaceutical company that uses proprietary technology to develop pharmaceutical proteins, including glycan-engineered monoclonal antibodies, biosimilars, follow-on biologics, and veterinary medicines (5). The LEX System process uses genetically transformed cultures of Lemna minor (duckweed) in manufacture of those products. As a plant-based production system, it addresses the need for control, containment, and cost reduction. The system has produced pharmaceutical compounds proven effective through phase 2 clinical studies. The current drug product made with LEX System technology (developed in conjunction with Netherlands-based Octoplus NV) is Locteron, a controlled-release interferon formulation for treating Hepatitis C (6,7,8).
Having used a relatively common species of aquatic monocot (Photo 1) to create a PMP manufacturing process, Biolex integrated its process into a single-use systems (SUS) approach. Adding SUS materials to the original PMP approach further reduced compliance concerns by nearly eliminating any need for cleaning validation upstream. Leveraging Single-Use Products: Benefits and Compliance
Combining PMP production processes with a new generation of single-use products presented an opportunity to the disposables industry. The synergies of those technologies became all the more compelling as requirements for plant genetic containment increased their hold on the PMP industry. In 2004, Biolex began testing bags for use as manufacturing culture vessels, biomass sequestering and filtration systems, and manifolds.
Each stage of the production process is designed to be closed and aseptic to build on the already animal-product-contact-free aspect of plant-based cultures. To further reduce cost and complexity, the design ensures that no classified areas are required for the upstream process. LEX System technology is designed to prevent contamination from all microorganisms and biocontaminants through the application of disposable aseptic connectors and gamma-sterilized single-use seed bags, production bags, harvest bags, and tubing assemblies. In addition, harvest has been simplified through application of a proprietary single-use design to create a fully disposable, low-cost material separation and sequestration step. With a tested and proven aseptic process, this technology stands exemplifies pharmaceutical process containment and control.
The single-use process addresses animal-product-free requirements, including extractables and leachables control through strict use of USP Class VI materials in the process stream. Using Class VI disposables hands off to SUS vendors a large part of the compliance, documentation, and regulatory control burden. Additional regulatory support is handled by in-house and external material and product testing, vendor auditing, and document management. All FDA and EMEA regulations pertaining to SU
S are strictly followed, and compliance control is built into the upstream process through a rigorous documentation and SOP management system.
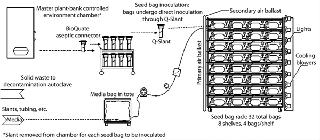
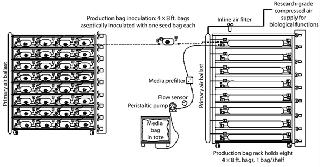
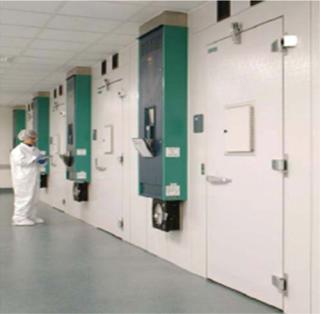
One of two distinct advantages of this production approach is that it transfers part of the disposables quality responsibility to vendors. The second advantage is that consistent application of single-use products throughout upstream processes eliminates all cleaning validation requirements for process stream hardware. That is a significant cost savings for Biolex and goes far toward offsetting the cost of disposables-based operations.
Regulatory Guidelines
Single-use regulatory guidelines cover bag materials in general and specific product-contact layers (11).
All Single-Use, GMP Materials
USP Class VI Cytotoxicity and Physicochemical Standards; USP Class VI Material Certificate, signed and dated (S&D).
Documentation with Certificate of Origin of Materials provided for all product contact materials; (S&D)
TSE, BSE free — for example, a statement should be provided that bovine-derived materials from bovine spongiform encephalopathy (BSE) countries or any products exposed to transmissible spongiform encephalopathy (TSE) agents (as defined by the US Department of Agriculture, 9 CFR 94.11) are not used or manipulated in the same facility as any of the materials of construction for the bag assembly (S&D)
All materials of construction must be animal-derived-component free (ADCF), (S&D)
Extractable and leachable screening results and documentation must be available as needed for pre- and poststerilization testing
Materials are gamma stable
Materials are UV stable
Additional Requirements for Product-Contact Layers
In addition to the above requirements, the following requirements are also applicable for bag product-contact layers:
Hemolysis ISO 1993-4 2002
Physicochemical USP28
Cytotoxicity MEM Elution, USP 28
Bacterial endotoxin; LAL <0.25 EU/mL extraction media, pass
Moisture permeability, E-96-90 method, <0.3 g/100 in 24 hours The LEX System Process
Lemna (commonly known as duckweed) is an aquatic monocotyledonous plant that thrives in the top 1 cm of still, freshwater systems. Several of its characteristics make it ideally suited for PMP production (4), including its
-
ability for clonal reproduction
-
high protein content
-
rapid growth rate
-
classification as a nonindustrialized plant, not a food crop
-
growth in a wide range of conditions
-
ability to produce secreted or tissue-bound products.
-
In addition, Lemna‘s process advantages include
-
viral inactivation steps are not required
-
scale has no effect on yield per unit weight or protein quality
-
no pesticides, herbicides, or antibiotics are used in production cultures.
Clonal reproduction simplifies line management, propagation, and the process feed stream. The absence of pollen or flowers simplifies genetic containment. Lemna‘s high total soluble protein (TSP) content provides higher expression and target protein concentrations per unit biomass than other PMP platforms (9). Another strategically advantageous characteristic is its culture growth rate. Lemna doubles its biomass every 36 hours under normal growth conditions, a doubling rate that exceeds other plant systems biomass build rates. Process Control
Photosynthesis converts sunlight to energy for cellular processes, in which carbon dioxide is converted to biomass and cellular products such as target transgenic proteins. Consequently, to exploit Lemna‘s growth rate and high levels of expression, cultures must receive appropriate amounts of light and filtered ambient air. Other important environmental parameters are media composition and temperature.
In processes, facilities, and hardware designs, only those four critical environmental factors are considered. This simplicity goes far toward limiting overall process and hardware design complexity and final facility costs. The LEX System upstream process is in definite contrast with complex photobioreactor systems that require monitoring of many physical indices, precision controls, and cleaning validation.
Design of experiment (DoE) software established critical ranges for environmental parameters. Studies tested ranges for yield, repeatability, and robustness. A study of failure mode and effects analysis (FMEA) used a scaled-down model system. That model addressed the range of possible failures, effects of each failure on product quality and yield, and critical points for process failure responses. Those process ranges and failure data provided process design standards and established system robustness. A scaled-up manufacturing system confirmed those results.
In addition to environmental testing, DoE addressing media composition, storage life, and postharvest FMEA added further critical guidelines for media preparation and vessel filling processes. Criteria for product storage were developed through HPLC quality analysis of storage regimes for harvested media and tissue. Those data were added to the overall process design to set hold-step requirements and facilitate raw-product transfer method controls.
Intensive collaboration among upstream process development, quality assurance (QA), and regulatory and operations functions throughout development of the production format was necessary to ensure minimized requirements for redesigns. This ensured careful considerations of regulations from the FDA and European agencies at all stages of development. For example, the potential for animal-product exposure was designed out of the process, as were any activities difficult to validate or hard to control and document. Lot definition requirements also played an important role in developing
the best and most compliant lot characterization.
Regulators have responded to this single-use, plant-based process with several comments and observations (10):
-
simplicity, few critical process parameters (CPP)
-
no animal-derived components in the process or media
-
no viral inactivation or prion clearance measures required
-
closed system: upstream processing can be in unclassified area
-
most special GMP guidances for transgenic plants do not apply
-
no open field production
-
no herbicides, pesticides, antibiotics, or environmental bioburden
-
no pollen or seeds due to clonal propagation.
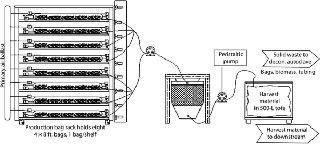
Single-Use Component Benefits and Requirements
This plant-based process uses proprietary culture bags designed to meet vertical and horizontal stacking density and scaling requirements. All filters, aseptic connectors, ports, and pressurized systems undergo extensive in-housing testing for baseline characteristics, flow volume and rate, burst pressure, barrier effectiveness, and product consistency.
The “Regulatory Guidelines” box provides an example of regulatory materials of construction (MoC) requirements for the disposable, production-scale vessels.As indicated in that list, animal-contact–free MoC are of primary importance. All products are certified animal-product–free before any purchases for GMP operations. Each approved item is shipped from its vendor with a certificate of authenticity (C of A), Certificate of conformity (C of C), lot number, and sterilization records.
One important component that helped Biolex design its enclosed, aseptic upstream process was the latest generation of aseptic connectors (Photo 2). When properly used, these connectors (BioQuate ReadyMate) are reported to provide an aseptic/sterile connection while requiring no classified areas or external sterile air-flow system.
Based on that design, process connections between bags, for example, could be made simply. Aseptic integrity of the components could be ensured despite gray-space operation. This type of aseptic connector proved a critical factor in lowering facility costs and simplifying process and hardware design. Due to their strategic role — and to prove compliance and control — it was necessary for those connectors to undergo a comprehensive testing regimen that included bacterial challenges, consistency testing, durability analysis, and additional FMEA testing. Process Flow
Upstream production begins with a master plant bank (MPB), the plant analogue of the more commonly known “master cell bank” (Photo 3). The LEX System process has demonstrated that small numbers of fronds can be stored in agar slants at 8–10 °C for up to a year with full viability and no genetic drift. To further control and preserve the plant bank, LEX System technology relies on a proprietary cryopreservation-based living line archival system. Although conventional archive systems have proven plant viability through nine years, cryopreservation systems have the potential to store recoverable plant material for much longer, possibly 20 years or more.
This upstream process begins when the contents of slants are transferred to “seed bags” (Photo 4, Figure 1). Once inoculated and supplied with media, the seed bags are placed on a lighted rack and attached to a compressed air supply. Other than temperature control through rack design and production-suite air handling, the only environmental support these cultures require are compressed air for biological processes and light for photosynthesis. The support rack is a proprietary, customized, self-contained lighting system with bag restraint, temperature control, and air distribution built in (Photo 5). Each rack can provide temperature feedback to the HVAC system for complete homeostatic control.
When the seed bag incubation cycle is nearly complete, in-process testing (IPT) samples are used to detail the integrity of the cultures. All bags with bioburden are removed from the process stream. Once seed-bag incubation is complete, those bags are connected to production bags through aseptic connectors (Figure 2). The contents of the seed bag are decanted into the production bag, and the connection between the two is sealed and cut (Figure 2). Used seed bags are deactivated through sterilization and processed for waste or materials recycling.
As production cultures reach maturity, samples are removed through the sample port and sent to QC for analysis. Bioburden-free bags continue in the process stream to harvest. Because the process is robust, contamination is very rare.
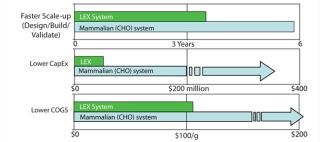
During harvest of the production bags, pumps move media and tissue through a proprietary, disposable “harvest bag” (Figure 3). While the harvest bag captures and encloses solid materials, media flow through the bag’s filtration panel and are pumped to a large collection tote for transfer to downstream processing. In a sealed single-use tubing network, the content of each bag is protected from contamination throughout the harvest process (as with the rest of the upstream process). If target proteins are secreted into the media, fronds sequestered in the harvest bag are decontaminated and discarded per USDA regulations. This production process requires none of the stirring or active control of media constituents, pH, or CO2 tension that many conventional batch processes require.
Once recovered, media are removed and sent downstream for clarification and additional purification into API. If a target protein is tissue bound, then harvest bags containing biomass and product are transferred to downstream processing. That involves simple disruption to extract protein from the biomass. Often a low-pH precipitation can significantly reduce host proteins and remove phenolic compounds. Depending on scale, either centrifugation or depth filtration clarifies the extract. Standard, relatively low-cost equipment is used for those steps, and no viral clearance is required. The rest of the downstream process is typical and depends on the target protein. For example, protein A capture is used for antibodies followed by one or two additional chromatography steps. Either biomass or media can be frozen and stored for later processing, if desired. Effective PMP System
LEX System technology offers several advantages over other systems (Figure 4). It minimizes the risk of accidental genetic release of product or contamination of food sources. Although nontoxic and edible, Lemna is not commonly used as a food source. A further advantage is that no pesticides, herbicides, or antibiotics are used in Lemna cultu
res. In addition, the single-use aspect of this production system has also nearly eliminated cleaning validation activities from upstream processes.
The current single-use process has handed off materials and product validation responsibility to vendors and manufacturers of disposable products. This has provided additional benefits of reduced work-force requirements and documentation burden.
Using disposable aseptic connectors eliminates the need for classified areas in upstream processes. This reduces facility and operating costs and process complexity. Aseptic connectors provided for a process that can specify and achieve low contamination levels, well within process requirements. In receiving a contaminant-free feed stream, downstream processing benefits as well.
LEX System technology has merged plant-based pharmaceutical protein production with single-use materials. The benefits and advantages to this approach have been demonstrated through investor support and FDA acknowledgement of successful clinical trials. A crucial synergy has manifested itself in the careful adaptation of single-use products to the Lemna-based PMP technology.
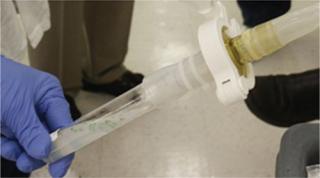
Photo 5:
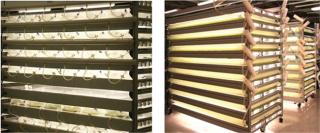
A Novel Approach
Biolex is a privately owned, clinical-stage biopharmaceutical company that uses proprietary technology to develop pharmaceutical proteins, including glycan-engineered monoclonal antibodies, biosimilars, follow-on biologics, and veterinary medicines (5). The LEX System process uses genetically transformed cultures of Lemna minor (duckweed) in manufacture of those products. As a plant-based production system, it addresses the need for control, containment, and cost reduction. The system has produced pharmaceutical compounds proven effective through phase 2 clinical studies. The current drug product made with LEX System technology (developed in conjunction with Netherlands-based Octoplus NV) is Locteron, a controlled-release interferon formulation for treating Hepatitis C (6,7,8).
Having used a relatively common species of aquatic monocot (Photo 1) to create a PMP manufacturing process, Biolex integrated its process into a single-use systems (SUS) approach. Adding SUS materials to the original PMP approach further reduced compliance concerns by nearly eliminating any need for cleaning validation upstream. Leveraging Single-Use Products: Benefits and Compliance
Combining PMP production processes with a new generation of single-use products presented an opportunity to the disposables industry. The synergies of those technologies became all the more compelling as requirements for plant genetic containment increased their hold on the PMP industry. In 2004, Biolex began testing bags for use as manufacturing culture vessels, biomass sequestering and filtration systems, and manifolds.
Each stage of the production process is designed to be closed and aseptic to build on the already animal-product-contact-free aspect of plant-based cultures. To further reduce cost and complexity, the design ensures that no classified areas are required for the upstream process. LEX System technology is designed to prevent contamination from all microorganisms and biocontaminants through the application of disposable aseptic connectors and gamma-sterilized single-use seed bags, production bags, harvest bags, and tubing assemblies. In addition, harvest has been simplified through application of a proprietary single-use design to create a fully disposable, low-cost material separation and sequestration step. With a tested and proven aseptic process, this technology stands exemplifies pharmaceutical process containment and control.
The single-use process addresses animal-product-free requirements, including extractables and leachables control through strict use of USP Class VI materials in the process stream. Using Class VI disposables hands off to SUS vendors a large part of the compliance, documentation, and regulatory control burden. Additional regulatory support is handled by in-house and external material and product testing, vendor auditing, and document management. All FDA and EMEA regulations pertaining to SU
S are strictly followed, and compliance control is built into the upstream process through a rigorous documentation and SOP management system.
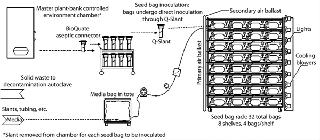
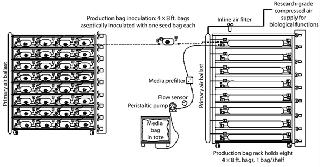
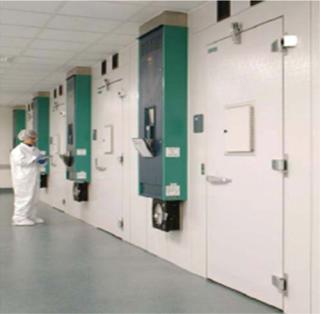
One of two distinct advantages of this production approach is that it transfers part of the disposables quality responsibility to vendors. The second advantage is that consistent application of single-use products throughout upstream processes eliminates all cleaning validation requirements for process stream hardware. That is a significant cost savings for Biolex and goes far toward offsetting the cost of disposables-based operations.
Regulatory Guidelines
Single-use regulatory guidelines cover bag materials in general and specific product-contact layers (11).
All Single-Use, GMP Materials
USP Class VI Cytotoxicity and Physicochemical Standards; USP Class VI Material Certificate, signed and dated (S&D).
Documentation with Certificate of Origin of Materials provided for all product contact materials; (S&D)
TSE, BSE free — for example, a statement should be provided that bovine-derived materials from bovine spongiform encephalopathy (BSE) countries or any products exposed to transmissible spongiform encephalopathy (TSE) agents (as defined by the US Department of Agriculture, 9 CFR 94.11) are not used or manipulated in the same facility as any of the materials of construction for the bag assembly (S&D)
All materials of construction must be animal-derived-component free (ADCF), (S&D)
Extractable and leachable screening results and documentation must be available as needed for pre- and poststerilization testing
Materials are gamma stable
Materials are UV stable
Additional Requirements for Product-Contact Layers
In addition to the above requirements, the following requirements are also applicable for bag product-contact layers:
Hemolysis ISO 1993-4 2002
Physicochemical USP28
Cytotoxicity MEM Elution, USP 28
Bacterial endotoxin; LAL <0.25 EU/mL extraction media, pass
Moisture permeability, E-96-90 method, <0.3 g/100 in 24 hours The LEX System Process
Lemna (commonly known as duckweed) is an aquatic monocotyledonous plant that thrives in the top 1 cm of still, freshwater systems. Several of its characteristics make it ideally suited for PMP production (4), including its
-
ability for clonal reproduction
-
high protein content
-
rapid growth rate
-
classification as a nonindustrialized plant, not a food crop
-
growth in a wide range of conditions
-
ability to produce secreted or tissue-bound products.
-
In addition, Lemna‘s process advantages include
-
viral inactivation steps are not required
-
scale has no effect on yield per unit weight or protein quality
-
no pesticides, herbicides, or antibiotics are used in production cultures.
Clonal reproduction simplifies line management, propagation, and the process feed stream. The absence of pollen or flowers simplifies genetic containment. Lemna‘s high total soluble protein (TSP) content provides higher expression and target protein concentrations per unit biomass than other PMP platforms (9). Another strategically advantageous characteristic is its culture growth rate. Lemna doubles its biomass every 36 hours under normal growth conditions, a doubling rate that exceeds other plant systems biomass build rates. Process Control
Photosynthesis converts sunlight to energy for cellular processes, in which carbon dioxide is converted to biomass and cellular products such as target transgenic proteins. Consequently, to exploit Lemna‘s growth rate and high levels of expression, cultures must receive appropriate amounts of light and filtered ambient air. Other important environmental parameters are media composition and temperature.
In processes, facilities, and hardware designs, only those four critical environmental factors are considered. This simplicity goes far toward limiting overall process and hardware design complexity and final facility costs. The LEX System upstream process is in definite contrast with complex photobioreactor systems that require monitoring of many physical indices, precision controls, and cleaning validation.
Design of experiment (DoE) software established critical ranges for environmental parameters. Studies tested ranges for yield, repeatability, and robustness. A study of failure mode and effects analysis (FMEA) used a scaled-down model system. That model addressed the range of possible failures, effects of each failure on product quality and yield, and critical points for process failure responses. Those process ranges and failure data provided process design standards and established system robustness. A scaled-up manufacturing system confirmed those results.
In addition to environmental testing, DoE addressing media composition, storage life, and postharvest FMEA added further critical guidelines for media preparation and vessel filling processes. Criteria for product storage were developed through HPLC quality analysis of storage regimes for harvested media and tissue. Those data were added to the overall process design to set hold-step requirements and facilitate raw-product transfer method controls.
Intensive collaboration among upstream process development, quality assurance (QA), and regulatory and operations functions throughout development of the production format was necessary to ensure minimized requirements for redesigns. This ensured careful considerations of regulations from the FDA and European agencies at all stages of development. For example, the potential for animal-product exposure was designed out of the process, as were any activities difficult to validate or hard to control and document. Lot definition requirements also played an important role in developing
the best and most compliant lot characterization.
Regulators have responded to this single-use, plant-based process with several comments and observations (10):
-
simplicity, few critical process parameters (CPP)
-
no animal-derived components in the process or media
-
no viral inactivation or prion clearance measures required
-
closed system: upstream processing can be in unclassified area
-
most special GMP guidances for transgenic plants do not apply
-
no open field production
-
no herbicides, pesticides, antibiotics, or environmental bioburden
-
no pollen or seeds due to clonal propagation.
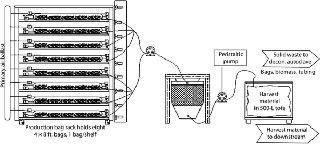
Single-Use Component Benefits and Requirements
This plant-based process uses proprietary culture bags designed to meet vertical and horizontal stacking density and scaling requirements. All filters, aseptic connectors, ports, and pressurized systems undergo extensive in-housing testing for baseline characteristics, flow volume and rate, burst pressure, barrier effectiveness, and product consistency.
The “Regulatory Guidelines” box provides an example of regulatory materials of construction (MoC) requirements for the disposable, production-scale vessels.As indicated in that list, animal-contact–free MoC are of primary importance. All products are certified animal-product–free before any purchases for GMP operations. Each approved item is shipped from its vendor with a certificate of authenticity (C of A), Certificate of conformity (C of C), lot number, and sterilization records.
One important component that helped Biolex design its enclosed, aseptic upstream process was the latest generation of aseptic connectors (Photo 2). When properly used, these connectors (BioQuate ReadyMate) are reported to provide an aseptic/sterile connection while requiring no classified areas or external sterile air-flow system.
Based on that design, process connections between bags, for example, could be made simply. Aseptic integrity of the components could be ensured despite gray-space operation. This type of aseptic connector proved a critical factor in lowering facility costs and simplifying process and hardware design. Due to their strategic role — and to prove compliance and control — it was necessary for those connectors to undergo a comprehensive testing regimen that included bacterial challenges, consistency testing, durability analysis, and additional FMEA testing. Process Flow
Upstream production begins with a master plant bank (MPB), the plant analogue of the more commonly known “master cell bank” (Photo 3). The LEX System process has demonstrated that small numbers of fronds can be stored in agar slants at 8–10 °C for up to a year with full viability and no genetic drift. To further control and preserve the plant bank, LEX System technology relies on a proprietary cryopreservation-based living line archival system. Although conventional archive systems have proven plant viability through nine years, cryopreservation systems have the potential to store recoverable plant material for much longer, possibly 20 years or more.
This upstream process begins when the contents of slants are transferred to “seed bags” (Photo 4, Figure 1). Once inoculated and supplied with media, the seed bags are placed on a lighted rack and attached to a compressed air supply. Other than temperature control through rack design and production-suite air handling, the only environmental support these cultures require are compressed air for biological processes and light for photosynthesis. The support rack is a proprietary, customized, self-contained lighting system with bag restraint, temperature control, and air distribution built in (Photo 5). Each rack can provide temperature feedback to the HVAC system for complete homeostatic control.
When the seed bag incubation cycle is nearly complete, in-process testing (IPT) samples are used to detail the integrity of the cultures. All bags with bioburden are removed from the process stream. Once seed-bag incubation is complete, those bags are connected to production bags through aseptic connectors (Figure 2). The contents of the seed bag are decanted into the production bag, and the connection between the two is sealed and cut (Figure 2). Used seed bags are deactivated through sterilization and processed for waste or materials recycling.
As production cultures reach maturity, samples are removed through the sample port and sent to QC for analysis. Bioburden-free bags continue in the process stream to harvest. Because the process is robust, contamination is very rare.
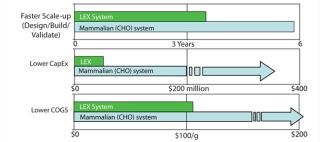
During harvest of the production bags, pumps move media and tissue through a proprietary, disposable “harvest bag” (Figure 3). While the harvest bag captures and encloses solid materials, media flow through the bag’s filtration panel and are pumped to a large collection tote for transfer to downstream processing. In a sealed single-use tubing network, the content of each bag is protected from contamination throughout the harvest process (as with the rest of the upstream process). If target proteins are secreted into the media, fronds sequestered in the harvest bag are decontaminated and discarded per USDA regulations. This production process requires none of the stirring or active control of media constituents, pH, or CO2 tension that many conventional batch processes require.
Once recovered, media are removed and sent downstream for clarification and additional purification into API. If a target protein is tissue bound, then harvest bags containing biomass and product are transferred to downstream processing. That involves simple disruption to extract protein from the biomass. Often a low-pH precipitation can significantly reduce host proteins and remove phenolic compounds. Depending on scale, either centrifugation or depth filtration clarifies the extract. Standard, relatively low-cost equipment is used for those steps, and no viral clearance is required. The rest of the downstream process is typical and depends on the target protein. For example, protein A capture is used for antibodies followed by one or two additional chromatography steps. Either biomass or media can be frozen and stored for later processing, if desired. Effective PMP System
LEX System technology offers several advantages over other systems (Figure 4). It minimizes the risk of accidental genetic release of product or contamination of food sources. Although nontoxic and edible, Lemna is not commonly used as a food source. A further advantage is that no pesticides, herbicides, or antibiotics are used in Lemna cultu
res. In addition, the single-use aspect of this production system has also nearly eliminated cleaning validation activities from upstream processes.
The current single-use process has handed off materials and product validation responsibility to vendors and manufacturers of disposable products. This has provided additional benefits of reduced work-force requirements and documentation burden.
Using disposable aseptic connectors eliminates the need for classified areas in upstream processes. This reduces facility and operating costs and process complexity. Aseptic connectors provided for a process that can specify and achieve low contamination levels, well within process requirements. In receiving a contaminant-free feed stream, downstream processing benefits as well.
LEX System technology has merged plant-based pharmaceutical protein production with single-use materials. The benefits and advantages to this approach have been demonstrated through investor support and FDA acknowledgement of successful clinical trials. A crucial synergy has manifested itself in the careful adaptation of single-use products to the Lemna-based PMP technology.
S are strictly followed, and compliance control is built into the upstream process through a rigorous documentation and SOP management system.
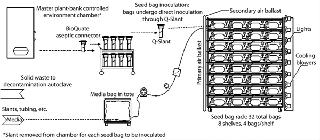
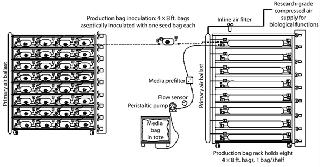
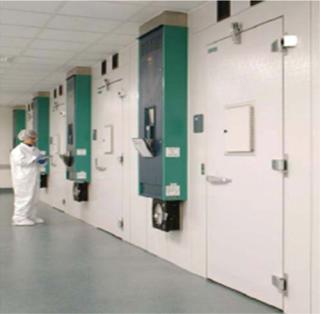
One of two distinct advantages of this production approach is that it transfers part of the disposables quality responsibility to vendors. The second advantage is that consistent application of single-use products throughout upstream processes eliminates all cleaning validation requirements for process stream hardware. That is a significant cost savings for Biolex and goes far toward offsetting the cost of disposables-based operations.
Regulatory Guidelines
Single-use regulatory guidelines cover bag materials in general and specific product-contact layers (11).
All Single-Use, GMP Materials
USP Class VI Cytotoxicity and Physicochemical Standards; USP Class VI Material Certificate, signed and dated (S&D).
Documentation with Certificate of Origin of Materials provided for all product contact materials; (S&D)
TSE, BSE free — for example, a statement should be provided that bovine-derived materials from bovine spongiform encephalopathy (BSE) countries or any products exposed to transmissible spongiform encephalopathy (TSE) agents (as defined by the US Department of Agriculture, 9 CFR 94.11) are not used or manipulated in the same facility as any of the materials of construction for the bag assembly (S&D)
All materials of construction must be animal-derived-component free (ADCF), (S&D)
Extractable and leachable screening results and documentation must be available as needed for pre- and poststerilization testing
Materials are gamma stable
Materials are UV stable
Additional Requirements for Product-Contact Layers
In addition to the above requirements, the following requirements are also applicable for bag product-contact layers:
Hemolysis ISO 1993-4 2002
Physicochemical USP28
Cytotoxicity MEM Elution, USP 28
Bacterial endotoxin; LAL <0.25 EU/mL extraction media, pass
Moisture permeability, E-96-90 method, <0.3 g/100 in 24 hours The LEX System Process
Lemna (commonly known as duckweed) is an aquatic monocotyledonous plant that thrives in the top 1 cm of still, freshwater systems. Several of its characteristics make it ideally suited for PMP production (4), including its
-
ability for clonal reproduction
-
high protein content
-
rapid growth rate
-
classification as a nonindustrialized plant, not a food crop
-
growth in a wide range of conditions
-
ability to produce secreted or tissue-bound products.
-
In addition, Lemna‘s process advantages include
-
viral inactivation steps are not required
-
scale has no effect on yield per unit weight or protein quality
-
no pesticides, herbicides, or antibiotics are used in production cultures.
Clonal reproduction simplifies line management, propagation, and the process feed stream. The absence of pollen or flowers simplifies genetic containment. Lemna‘s high total soluble protein (TSP) content provides higher expression and target protein concentrations per unit biomass than other PMP platforms (9). Another strategically advantageous characteristic is its culture growth rate. Lemna doubles its biomass every 36 hours under normal growth conditions, a doubling rate that exceeds other plant systems biomass build rates. Process Control
Photosynthesis converts sunlight to energy for cellular processes, in which carbon dioxide is converted to biomass and cellular products such as target transgenic proteins. Consequently, to exploit Lemna‘s growth rate and high levels of expression, cultures must receive appropriate amounts of light and filtered ambient air. Other important environmental parameters are media composition and temperature.
In processes, facilities, and hardware designs, only those four critical environmental factors are considered. This simplicity goes far toward limiting overall process and hardware design complexity and final facility costs. The LEX System upstream process is in definite contrast with complex photobioreactor systems that require monitoring of many physical indices, precision controls, and cleaning validation.
Design of experiment (DoE) software established critical ranges for environmental parameters. Studies tested ranges for yield, repeatability, and robustness. A study of failure mode and effects analysis (FMEA) used a scaled-down model system. That model addressed the range of possible failures, effects of each failure on product quality and yield, and critical points for process failure responses. Those process ranges and failure data provided process design standards and established system robustness. A scaled-up manufacturing system confirmed those results.
In addition to environmental testing, DoE addressing media composition, storage life, and postharvest FMEA added further critical guidelines for media preparation and vessel filling processes. Criteria for product storage were developed through HPLC quality analysis of storage regimes for harvested media and tissue. Those data were added to the overall process design to set hold-step requirements and facilitate raw-product transfer method controls.
Intensive collaboration among upstream process development, quality assurance (QA), and regulatory and operations functions throughout development of the production format was necessary to ensure minimized requirements for redesigns. This ensured careful considerations of regulations from the FDA and European agencies at all stages of development. For example, the potential for animal-product exposure was designed out of the process, as were any activities difficult to validate or hard to control and document. Lot definition requirements also played an important role in developing
the best and most compliant lot characterization.
Regulators have responded to this single-use, plant-based process with several comments and observations (10):
-
simplicity, few critical process parameters (CPP)
-
no animal-derived components in the process or media
-
no viral inactivation or prion clearance measures required
-
closed system: upstream processing can be in unclassified area
-
most special GMP guidances for transgenic plants do not apply
-
no open field production
-
no herbicides, pesticides, antibiotics, or environmental bioburden
-
no pollen or seeds due to clonal propagation.
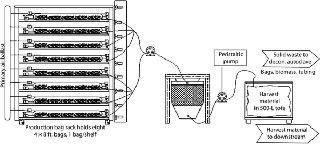
Single-Use Component Benefits and Requirements
This plant-based process uses proprietary culture bags designed to meet vertical and horizontal stacking density and scaling requirements. All filters, aseptic connectors, ports, and pressurized systems undergo extensive in-housing testing for baseline characteristics, flow volume and rate, burst pressure, barrier effectiveness, and product consistency.
The “Regulatory Guidelines” box provides an example of regulatory materials of construction (MoC) requirements for the disposable, production-scale vessels.As indicated in that list, animal-contact–free MoC are of primary importance. All products are certified animal-product–free before any purchases for GMP operations. Each approved item is shipped from its vendor with a certificate of authenticity (C of A), Certificate of conformity (C of C), lot number, and sterilization records.
One important component that helped Biolex design its enclosed, aseptic upstream process was the latest generation of aseptic connectors (Photo 2). When properly used, these connectors (BioQuate ReadyMate) are reported to provide an aseptic/sterile connection while requiring no classified areas or external sterile air-flow system.
Based on that design, process connections between bags, for example, could be made simply. Aseptic integrity of the components could be ensured despite gray-space operation. This type of aseptic connector proved a critical factor in lowering facility costs and simplifying process and hardware design. Due to their strategic role — and to prove compliance and control — it was necessary for those connectors to undergo a comprehensive testing regimen that included bacterial challenges, consistency testing, durability analysis, and additional FMEA testing. Process Flow
Upstream production begins with a master plant bank (MPB), the plant analogue of the more commonly known “master cell bank” (Photo 3). The LEX System process has demonstrated that small numbers of fronds can be stored in agar slants at 8–10 °C for up to a year with full viability and no genetic drift. To further control and preserve the plant bank, LEX System technology relies on a proprietary cryopreservation-based living line archival system. Although conventional archive systems have proven plant viability through nine years, cryopreservation systems have the potential to store recoverable plant material for much longer, possibly 20 years or more.
This upstream process begins when the contents of slants are transferred to “seed bags” (Photo 4, Figure 1). Once inoculated and supplied with media, the seed bags are placed on a lighted rack and attached to a compressed air supply. Other than temperature control through rack design and production-suite air handling, the only environmental support these cultures require are compressed air for biological processes and light for photosynthesis. The support rack is a proprietary, customized, self-contained lighting system with bag restraint, temperature control, and air distribution built in (Photo 5). Each rack can provide temperature feedback to the HVAC system for complete homeostatic control.
When the seed bag incubation cycle is nearly complete, in-process testing (IPT) samples are used to detail the integrity of the cultures. All bags with bioburden are removed from the process stream. Once seed-bag incubation is complete, those bags are connected to production bags through aseptic connectors (Figure 2). The contents of the seed bag are decanted into the production bag, and the connection between the two is sealed and cut (Figure 2). Used seed bags are deactivated through sterilization and processed for waste or materials recycling.
As production cultures reach maturity, samples are removed through the sample port and sent to QC for analysis. Bioburden-free bags continue in the process stream to harvest. Because the process is robust, contamination is very rare.
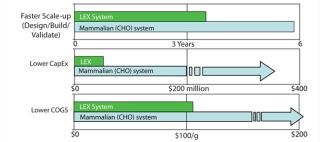
During harvest of the production bags, pumps move media and tissue through a proprietary, disposable “harvest bag” (Figure 3). While the harvest bag captures and encloses solid materials, media flow through the bag’s filtration panel and are pumped to a large collection tote for transfer to downstream processing. In a sealed single-use tubing network, the content of each bag is protected from contamination throughout the harvest process (as with the rest of the upstream process). If target proteins are secreted into the media, fronds sequestered in the harvest bag are decontaminated and discarded per USDA regulations. This production process requires none of the stirring or active control of media constituents, pH, or CO2 tension that many conventional batch processes require.
Once recovered, media are removed and sent downstream for clarification and additional purification into API. If a target protein is tissue bound, then harvest bags containing biomass and product are transferred to downstream processing. That involves simple disruption to extract protein from the biomass. Often a low-pH precipitation can significantly reduce host proteins and remove phenolic compounds. Depending on scale, either centrifugation or depth filtration clarifies the extract. Standard, relatively low-cost equipment is used for those steps, and no viral clearance is required. The rest of the downstream process is typical and depends on the target protein. For example, protein A capture is used for antibodies followed by one or two additional chromatography steps. Either biomass or media can be frozen and stored for later processing, if desired. Effective PMP System
LEX System technology offers several advantages over other systems (Figure 4). It minimizes the risk of accidental genetic release of product or contamination of food sources. Although nontoxic and edible, Lemna is not commonly used as a food source. A further advantage is that no pesticides, herbicides, or antibiotics are used in Lemna cultu
res. In addition, the single-use aspect of this production system has also nearly eliminated cleaning validation activities from upstream processes.
The current single-use process has handed off materials and product validation responsibility to vendors and manufacturers of disposable products. This has provided additional benefits of reduced work-force requirements and documentation burden.
Using disposable aseptic connectors eliminates the need for classified areas in upstream processes. This reduces facility and operating costs and process complexity. Aseptic connectors provided for a process that can specify and achieve low contamination levels, well within process requirements. In receiving a contaminant-free feed stream, downstream processing benefits as well.
LEX System technology has merged plant-based pharmaceutical protein production with single-use materials. The benefits and advantages to this approach have been demonstrated through investor support and FDA acknowledgement of successful clinical trials. A crucial synergy has manifested itself in the careful adaptation of single-use products to the Lemna-based PMP technology.
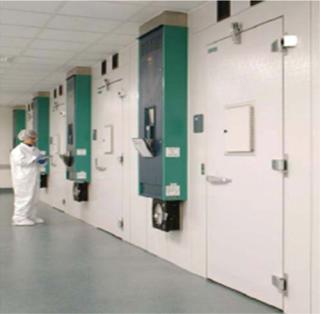
One of two distinct advantages of this production approach is that it transfers part of the disposables quality responsibility to vendors. The second advantage is that consistent application of single-use products throughout upstream processes eliminates all cleaning validation requirements for process stream hardware. That is a significant cost savings for Biolex and goes far toward offsetting the cost of disposables-based operations.
Regulatory Guidelines
Single-use regulatory guidelines cover bag materials in general and specific product-contact layers (11).
All Single-Use, GMP Materials
USP Class VI Cytotoxicity and Physicochemical Standards; USP Class VI Material Certificate, signed and dated (S&D).
Documentation with Certificate of Origin of Materials provided for all product contact materials; (S&D)
TSE, BSE free — for example, a statement should be provided that bovine-derived materials from bovine spongiform encephalopathy (BSE) countries or any products exposed to transmissible spongiform encephalopathy (TSE) agents (as defined by the US Department of Agriculture, 9 CFR 94.11) are not used or manipulated in the same facility as any of the materials of construction for the bag assembly (S&D)
All materials of construction must be animal-derived-component free (ADCF), (S&D)
Extractable and leachable screening results and documentation must be available as needed for pre- and poststerilization testing
Materials are gamma stable
Materials are UV stable
Additional Requirements for Product-Contact Layers
In addition to the above requirements, the following requirements are also applicable for bag product-contact layers:
Hemolysis ISO 1993-4 2002
Physicochemical USP28
Cytotoxicity MEM Elution, USP 28
Bacterial endotoxin; LAL <0.25 EU/mL extraction media, pass
Moisture permeability, E-96-90 method, <0.3 g/100 in 24 hours The LEX System Process
Lemna (commonly known as duckweed) is an aquatic monocotyledonous plant that thrives in the top 1 cm of still, freshwater systems. Several of its characteristics make it ideally suited for PMP production (4), including its
-
ability for clonal reproduction
-
high protein content
-
rapid growth rate
-
classification as a nonindustrialized plant, not a food crop
-
growth in a wide range of conditions
-
ability to produce secreted or tissue-bound products.
-
In addition, Lemna‘s process advantages include
-
viral inactivation steps are not required
-
scale has no effect on yield per unit weight or protein quality
-
no pesticides, herbicides, or antibiotics are used in production cultures.
Clonal reproduction simplifies line management, propagation, and the process feed stream. The absence of pollen or flowers simplifies genetic containment. Lemna‘s high total soluble protein (TSP) content provides higher expression and target protein concentrations per unit biomass than other PMP platforms (9). Another strategically advantageous characteristic is its culture growth rate. Lemna doubles its biomass every 36 hours under normal growth conditions, a doubling rate that exceeds other plant systems biomass build rates. Process Control
Photosynthesis converts sunlight to energy for cellular processes, in which carbon dioxide is converted to biomass and cellular products such as target transgenic proteins. Consequently, to exploit Lemna‘s growth rate and high levels of expression, cultures must receive appropriate amounts of light and filtered ambient air. Other important environmental parameters are media composition and temperature.
In processes, facilities, and hardware designs, only those four critical environmental factors are considered. This simplicity goes far toward limiting overall process and hardware design complexity and final facility costs. The LEX System upstream process is in definite contrast with complex photobioreactor systems that require monitoring of many physical indices, precision controls, and cleaning validation.
Design of experiment (DoE) software established critical ranges for environmental parameters. Studies tested ranges for yield, repeatability, and robustness. A study of failure mode and effects analysis (FMEA) used a scaled-down model system. That model addressed the range of possible failures, effects of each failure on product quality and yield, and critical points for process failure responses. Those process ranges and failure data provided process design standards and established system robustness. A scaled-up manufacturing system confirmed those results.
In addition to environmental testing, DoE addressing media composition, storage life, and postharvest FMEA added further critical guidelines for media preparation and vessel filling processes. Criteria for product storage were developed through HPLC quality analysis of storage regimes for harvested media and tissue. Those data were added to the overall process design to set hold-step requirements and facilitate raw-product transfer method controls.
Intensive collaboration among upstream process development, quality assurance (QA), and regulatory and operations functions throughout development of the production format was necessary to ensure minimized requirements for redesigns. This ensured careful considerations of regulations from the FDA and European agencies at all stages of development. For example, the potential for animal-product exposure was designed out of the process, as were any activities difficult to validate or hard to control and document. Lot definition requirements also played an important role in developing
the best and most compliant lot characterization.
Regulators have responded to this single-use, plant-based process with several comments and observations (10):
-
simplicity, few critical process parameters (CPP)
-
no animal-derived components in the process or media
-
no viral inactivation or prion clearance measures required
-
closed system: upstream processing can be in unclassified area
-
most special GMP guidances for transgenic plants do not apply
-
no open field production
-
no herbicides, pesticides, antibiotics, or environmental bioburden
-
no pollen or seeds due to clonal propagation.
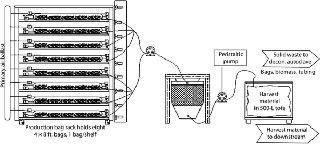
Single-Use Component Benefits and Requirements
This plant-based process uses proprietary culture bags designed to meet vertical and horizontal stacking density and scaling requirements. All filters, aseptic connectors, ports, and pressurized systems undergo extensive in-housing testing for baseline characteristics, flow volume and rate, burst pressure, barrier effectiveness, and product consistency.
The “Regulatory Guidelines” box provides an example of regulatory materials of construction (MoC) requirements for the disposable, production-scale vessels.As indicated in that list, animal-contact–free MoC are of primary importance. All products are certified animal-product–free before any purchases for GMP operations. Each approved item is shipped from its vendor with a certificate of authenticity (C of A), Certificate of conformity (C of C), lot number, and sterilization records.
One important component that helped Biolex design its enclosed, aseptic upstream process was the latest generation of aseptic connectors (Photo 2). When properly used, these connectors (BioQuate ReadyMate) are reported to provide an aseptic/sterile connection while requiring no classified areas or external sterile air-flow system.
Based on that design, process connections between bags, for example, could be made simply. Aseptic integrity of the components could be ensured despite gray-space operation. This type of aseptic connector proved a critical factor in lowering facility costs and simplifying process and hardware design. Due to their strategic role — and to prove compliance and control — it was necessary for those connectors to undergo a comprehensive testing regimen that included bacterial challenges, consistency testing, durability analysis, and additional FMEA testing. Process Flow
Upstream production begins with a master plant bank (MPB), the plant analogue of the more commonly known “master cell bank” (Photo 3). The LEX System process has demonstrated that small numbers of fronds can be stored in agar slants at 8–10 °C for up to a year with full viability and no genetic drift. To further control and preserve the plant bank, LEX System technology relies on a proprietary cryopreservation-based living line archival system. Although conventional archive systems have proven plant viability through nine years, cryopreservation systems have the potential to store recoverable plant material for much longer, possibly 20 years or more.
This upstream process begins when the contents of slants are transferred to “seed bags” (Photo 4, Figure 1). Once inoculated and supplied with media, the seed bags are placed on a lighted rack and attached to a compressed air supply. Other than temperature control through rack design and production-suite air handling, the only environmental support these cultures require are compressed air for biological processes and light for photosynthesis. The support rack is a proprietary, customized, self-contained lighting system with bag restraint, temperature control, and air distribution built in (Photo 5). Each rack can provide temperature feedback to the HVAC system for complete homeostatic control.
When the seed bag incubation cycle is nearly complete, in-process testing (IPT) samples are used to detail the integrity of the cultures. All bags with bioburden are removed from the process stream. Once seed-bag incubation is complete, those bags are connected to production bags through aseptic connectors (Figure 2). The contents of the seed bag are decanted into the production bag, and the connection between the two is sealed and cut (Figure 2). Used seed bags are deactivated through sterilization and processed for waste or materials recycling.
As production cultures reach maturity, samples are removed through the sample port and sent to QC for analysis. Bioburden-free bags continue in the process stream to harvest. Because the process is robust, contamination is very rare.
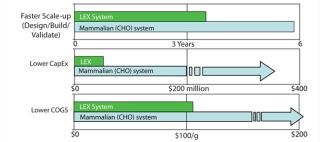
During harvest of the production bags, pumps move media and tissue through a proprietary, disposable “harvest bag” (Figure 3). While the harvest bag captures and encloses solid materials, media flow through the bag’s filtration panel and are pumped to a large collection tote for transfer to downstream processing. In a sealed single-use tubing network, the content of each bag is protected from contamination throughout the harvest process (as with the rest of the upstream process). If target proteins are secreted into the media, fronds sequestered in the harvest bag are decontaminated and discarded per USDA regulations. This production process requires none of the stirring or active control of media constituents, pH, or CO2 tension that many conventional batch processes require.
Once recovered, media are removed and sent downstream for clarification and additional purification into API. If a target protein is tissue bound, then harvest bags containing biomass and product are transferred to downstream processing. That involves simple disruption to extract protein from the biomass. Often a low-pH precipitation can significantly reduce host proteins and remove phenolic compounds. Depending on scale, either centrifugation or depth filtration clarifies the extract. Standard, relatively low-cost equipment is used for those steps, and no viral clearance is required. The rest of the downstream process is typical and depends on the target protein. For example, protein A capture is used for antibodies followed by one or two additional chromatography steps. Either biomass or media can be frozen and stored for later processing, if desired. Effective PMP System
LEX System technology offers several advantages over other systems (Figure 4). It minimizes the risk of accidental genetic release of product or contamination of food sources. Although nontoxic and edible, Lemna is not commonly used as a food source. A further advantage is that no pesticides, herbicides, or antibiotics are used in Lemna cultu
res. In addition, the single-use aspect of this production system has also nearly eliminated cleaning validation activities from upstream processes.
The current single-use process has handed off materials and product validation responsibility to vendors and manufacturers of disposable products. This has provided additional benefits of reduced work-force requirements and documentation burden.
Using disposable aseptic connectors eliminates the need for classified areas in upstream processes. This reduces facility and operating costs and process complexity. Aseptic connectors provided for a process that can specify and achieve low contamination levels, well within process requirements. In receiving a contaminant-free feed stream, downstream processing benefits as well.
LEX System technology has merged plant-based pharmaceutical protein production with single-use materials. The benefits and advantages to this approach have been demonstrated through investor support and FDA acknowledgement of successful clinical trials. A crucial synergy has manifested itself in the careful adaptation of single-use products to the Lemna-based PMP technology.
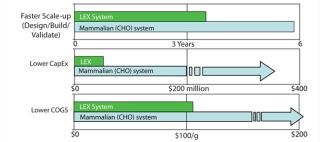
During harvest of the production bags, pumps move media and tissue through a proprietary, disposable “harvest bag” (Figure 3). While the harvest bag captures and encloses solid materials, media flow through the bag’s filtration panel and are pumped to a large collection tote for transfer to downstream processing. In a sealed single-use tubing network, the content of each bag is protected from contamination throughout the harvest process (as with the rest of the upstream process). If target proteins are secreted into the media, fronds sequestered in the harvest bag are decontaminated and discarded per USDA regulations. This production process requires none of the stirring or active control of media constituents, pH, or CO2 tension that many conventional batch processes require.
Once recovered, media are removed and sent downstream for clarification and additional purification into API. If a target protein is tissue bound, then harvest bags containing biomass and product are transferred to downstream processing. That involves simple disruption to extract protein from the biomass. Often a low-pH precipitation can significantly reduce host proteins and remove phenolic compounds. Depending on scale, either centrifugation or depth filtration clarifies the extract. Standard, relatively low-cost equipment is used for those steps, and no viral clearance is required. The rest of the downstream process is typical and depends on the target protein. For example, protein A capture is used for antibodies followed by one or two additional chromatography steps. Either biomass or media can be frozen and stored for later processing, if desired. Effective PMP System
LEX System technology offers several advantages over other systems (Figure 4). It minimizes the risk of accidental genetic release of product or contamination of food sources. Although nontoxic and edible, Lemna is not commonly used as a food source. A further advantage is that no pesticides, herbicides, or antibiotics are used in Lemna cultu
res. In addition, the single-use aspect of this production system has also nearly eliminated cleaning validation activities from upstream processes.
The current single-use process has handed off materials and product validation responsibility to vendors and manufacturers of disposable products. This has provided additional benefits of reduced work-force requirements and documentation burden.
Using disposable aseptic connectors eliminates the need for classified areas in upstream processes. This reduces facility and operating costs and process complexity. Aseptic connectors provided for a process that can specify and achieve low contamination levels, well within process requirements. In receiving a contaminant-free feed stream, downstream processing benefits as well.
LEX System technology has merged plant-based pharmaceutical protein production with single-use materials. The benefits and advantages to this approach have been demonstrated through investor support and FDA acknowledgement of successful clinical trials. A crucial synergy has manifested itself in the careful adaptation of single-use products to the Lemna-based PMP technology.
Author Details
Corresponding author Keith Everett is director of process design and research at Biolex Therapeutics, Inc, 158 Credle St., Pittsboro, NCNC 27312; 1-919-542-9901, ext. 2032; keverett@biolex.com. Lynn Dickey, PhD is vice president of research and technology development, John Parsons is research scientist of plant biology, Rachel Loranger is upstream research coordinator, and Vincent Wingate is associate director of upstream process development and plant biology, all at Biolex Therapeutics.
REFERENCES
1.) Office of the Press Secretary 2011.. The White House.
2.). Molecular Farming: Plant Bioreactors. 3.) Thomson, JA. 2006.Seeds for the Future: “The Impact of Genetically Modified Crops on the Environment, Cornell University Press, Ithaca. 4.) Gasdaska, JR, D Spencer, and L. Dickey. 2003. Advantages of Therapeutic Protein Production in the Aquatic Plant Lemna. BioProcessing J:49-56. 5.) Cox, KM. 2006. Glycan Optimization of a Human Monoclonal Antibody in the Aquatic Plant Lemna minor. Nature Biotech 24:1591-1597. 6.) Dzublyk, I. 2007. Phase 2a Study to Evaluate the Safety and Tolerability and Antiviral Effect of Four Doses of a Novel, Controlled-Release Interferon alfa-2b (LocteronTM) Given Every Two Weeks for 12 Weeks in Treatment-Naïve Patients with Chronic Hepatitis C (Genotype 1). J. Hepatology 46:4S:232A. 7.) Lawitz, E. 2010. Early Viral Response of Controlled-Release Interferon alpha2b and Ribavirin vs. Pegylated Interferon alpha 2b and Ribavirin in Treatment-Naïve Genotype1 Hepatitis C: 12 Week Results (SELECT-2 Trial). J. Hepatology 52:S114. 8.) Long, WA. 2011. Timing and Frequency of Depression During HCV-Treatment with Controlled Release INFa2b (CR2b) vs. Pegylated IFNa2b (PEG2b): Results from SELECT-2, a Randomized Open-Label 72-Week Comparison in 116 Treatment-Naïve Patients with Genotype 1 HCV. J. Hepatology 54:S181-182. 9.) Cox, KM. An, Z and W 2009.Production of Antibodies in PlantsTherapeutic Antibodies: From Theory to Practice, Wiley, Inc, New York. 10.) Private communication between Biolex and regulatory authorities. 11.) Biolex internal documents.