Regenerative medicine includes both cell and gene therapies. Currently 672 regenerative medicine companies operate around the world, and 20 products have been approved by the US Food and Drug Administration (FDA). Of 631 ongoing clinical trials by the end of 2015 (1), over 40% are in oncology, followed in prominence by cardiovascular and infectious diseases. Here I focus on gene and cell therapy bioprocessing in which the final products delivered to patients are cells.
Cell therapies are either autologous (derived from a single patient, for that patient) or allogeneic (coming from a banked donor source, for many patients). The former do not face risks associated with cell rejection; however, they are much more expensive to manufacture and distribute than the latter. Although Dendreon’s Provenge autologous cell therapy was approved by the FDA, it ultimately failed commercially because of its high cost to patients due to a manufacturing and distribution model that was inefficient. Cost of goods (CoG), manufacturing processes, and logistics are all critical to successful cell therapy commercialization, so they need to be considered along with clinical science from the inception of a cell therapy company. Three key enablers for success are manufacturing automation and single-use technologies; a diverse pipeline in modularized facilities; and sophisticated data acquisition and logistics.
Addressing Risk with Quality by Design (QbD)
Manufacturing cell therapy products typically requires many or all of the following steps: acquisition or generation of starting cells; cultivation, expansion, and modification of cells; harvest and cell concentration; purification; formulation, fill, and finish; and storage and shipping of the final product (2). Allogeneic cells are manufactured in a standardized process at a central facility before distribution.
For autologous therapies, more than one manufacturing site is involved:
- Cells are derived from a patient at a clinical site, then processed and returned to the patient at that site.
- Or cells are extracted and shipped to a central processing facility, then processed and shipped back to the patient.
The FDA requires cell therapy developers to demonstrate that no manufacturing changes affect their product profiles for safety, identity, purity, or potency (2). Comparability risk comes when a company needs to adjust its manufacturing process for commercial production after clinical trials have finished or during phase 3 under a different protocol. For instance, a major comparability risk would come from changing a critical raw material in a core process step (e.g., transitioning from animal serum to a serum-free culture medium). However, switching from manual to electronic record-keeping poses minimal risk.
A common method for testing comparability in regenerative medicine is to split a patient’s cells into two volumes and process both samples for comparison in parallel. Often, patient cells are unavailable, so normal donor cells must be used. In such cases, patient and donor cells should be shown to be equivalent through experimentation. If a major change is made, then a further clinical trial may be required to characterize its effect on the product profile. The risk of major manufacturing changes can be reduced by implementing a quality by design (QbD) approach to product development from the discovery stage.
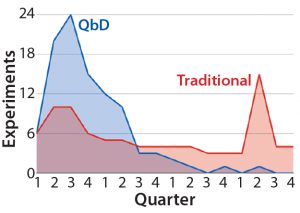
Figure 1: Distribution of experimental workload from initiation of a hypothetical cell-therapy manufacturing process development to completion — comparing traditional and QbD approaches (4)
QbD is a scientific risk-assessment framework for process design based on relating product and process attributes to product quality (3). Its first step is to identify a quality target product profile (QTPP), which includes the therapy type (autologous or allogeneic) and expected genotype, cell identity, required final-product viability, desired potency, the quantity of cells needed, identification of impurities that must be eliminated, and requirements for storage and stability. Determining relevant critical quality attributes (CQAs), critical process parameters (CPPs), and material attributes requires experimentation, mechanistic modeling, literature review, and application of a broad array of analytical techniques. Those activities would constitute the discovery phase of a given cell therapy. QbD helps companies prioritize their studies to the most influential critical process parameters and material attributes. In addition to reducing risk, QbD also increases efficiency by front-loading critical experiments (Figure 1). That is particularly important for early identification of process changes that might need to be made later on.
Improving Efficiency, Reducing Costs
Traditional academic discovery relies heavily on T-flasks for both adherent and non-adherent cell cultures. However, adopting a QbD approach to minimize future comparability risks suggests that discovery should involve culture vessels such as disposable bioreactors that match as closely as possible the target scaled-up process to be used in final current good manufacturing practice (CGMP) production. Disposable bioreactors are also feasible for one-patient–one-bioreactor operations. With the addition of microcarriers, bioreactors are appropriate for both adherent and nonadherent cell types. A number of such bioreactors are commercially available: e.g., Pall Life Sciences’ PadReactor system and XRS 20 bioreactor system, Thermo Scientific’s S.U.B. single-use bioreactors, GE Healthcare’s WAVE bioreactor, Millipore’s Mobius system, and Sartorius-Stedim Biotech’s BIOSTAT STR bioreactor.
Single-use technologies offer many advantages, including consistency in results from batch to batch; simplified handling for operators; savings of time, energy, and water; and dramatically decreased cross-contamination risks (5). Thus, disposable systems are of great interest, particularly because they reduce initial capital investment and the process footprint as well as costs linked to facility design itself (6). Footprint reduction at commercial scale is critical for the success of autologous cell therapies. Contained systems support automated cell expansion and purification instead of requiring dedicated classified space. Under monitored and controlled conditions in an integrated facility based on multiplexed, single-use bioreactors, production of final autologous products in multiple doses from starting cell populations donated by single patients is possible.
CoG analysis is critical to the success of all life-science commercialization. It requires an examination of direct costs, overhead costs, depreciation, and failed-batch costs (Table 1). This is particularly important for personalized/autologous cell therapies, which cannot benefit from economies of scale (7).
A major material cost for the cell therapy industry comes from bovine serum, which is routinely used in cell culture. As commercial-scale cell production facilities increase in number, the world supply of serum will become limiting to cell therapy manufacturing (8). Therefore, developing a manufacturing process independent of serum is a sensible consideration for nascent cell therapy companies to prevent future problems associated with serum shortage.
Another major direct cost is shipping. Most cellular therapies are shipped and distributed under at least –20 °C conditions. New technologies that allow higher temperature shipping are becoming available. The World Health Organization (WHO) dictates that distribution of pharmaceutical products must be conducted in compliance with CGMP, good storage practice (GSP), and good distribution practice (GDP) (9). Companies are obliged to make use of technologies such as temperature monitors during shipping and need contingency plans for temperature deviations beyond acceptable levels. Shipping and distribution costs can account for over 25% of regenerative medicines’ product cost (10). This should be considered during QbD planning.
In addition to minimizing cost of materials, QbD optimization and automation of a manufacturing production facility can reduce overhead costs. Because autologous cell processes depend on individual patients, associated production facilities can be idle periodically, which represents a major cost. So cell therapy companies need to integrate a portfolio of scalable and predictable products such as allogeneic cell therapies or cellular cancer vaccines that are compatible with the available autologous cell manufacturing space.
According to QbD, manufacturing capacity should be considered from the outset, along with the possibility of operating a mixed production facility through the use of contained systems such as disposable bioreactors to maximize output. Modular facilities can help companies expand or contract certain manufacturing processes as demand changes. That requires sophisticated logistics to track and predict capacity requirements for a given facility. If diversification of an autologous product portfolio is not possible, then it may be worth considering a contract manufacturing organization (CMO) that already uses modular systems. That could provide a major cost savings compared with a wholly owned but frequently idle single-product manufacturing facility.
Novel Analytical Technologies: Process analytical technologies (PAT) that allow for real-time monitoring of bioreactor conditions apply both to process development and quality assurance during final manufacture. The ability to monitor bioreactors inline and collect data wirelessly without direct sampling and operator intervention provides a significant cost savings. Inline monitoring also allows a system to remain closed, preventing contamination and fluctuations in temperature and pH.
Cell viability is critical for cell therapy. Real-time inline monitoring of glucose, dissolved oxygen (DO), pH, and temperature of cell growth medium in a bioreactor has been available for some time (11). The radio-frequency impedance method (capacitance) is frequently used to monitor cell viability in bioreactors inline (12). In addition, real-time automated monitoring of cell viability is now possible to measure the proportion of viable, early apoptotic, late apoptotic, and necrotic cell populations in bioreactors using a flow-cytometry method (13). Data from such outputs can be monitored by software algorithms that make real-time adjustments to keep bioreactor conditions optimized for cell viability. Automatic data collection also assists in monitoring batch-to-batch comparability, for which software can synthesize data from an entire manufacturing process.
Clearly the input of data scientists is critical during the design of automated data collection systems and development of appropriate algorithms. Consideration of data servers and information security is also important.
Process Scale-Up and Transfer: Small-scale (2–15 mL) bioreactors are ideal for process development. However, certain factors need to be taken into consideration for scale-up: e.g., identification of relevant scaling parameters, conversion of impeller geometry or mixing patterns to large-scale geometries, conversion of liquid-handling protocols to large-scale handling, development of appropriate sensors, and accounting for sparging (which is absent at small scales). Once these considerations have been taken into account, developers can choose from bioreactors available at a range of scales: <30 mL, <30 mL to 2 L, 2–100 L, and >100 L.
Modularization: Industrialization of autologous and allogeneic cell therapies is facilitated by modular manufacturing facilities. Genentech, Lilly, and Merck all use such facilities (14). Single -use equipment lacks some flexibility in a traditional facility; however, modular systems combined with single-use systems could be ideal for CMOs specializing in regenerative medicine with different volumes and processes for individual clients. Single-use systems shorten turnover time and increase a company’s flexibility in production planning. If capacity demands change, then a CMO can add or subtract some cleanroom areas.
Prefabricated cleanroom pods can be designed and built off-site. Once assembled into a facility, pods are linked for access through air locks. These pods can be used for an entire process or just a single step (14). This concept is potentially suitable for expanding autologous therapy manufacturing across hospital locations.
Improve your Chances of Success
Commercialization of cell and gene therapies can be enabled by adopting a QbD approach to manufacturing bioprocess development. Cost efficiencies can be realized with single-use bioreactors integrated into multiproduct pipeline automated modular facilities. Scale-up can be facilitated by QbD planning, considering serum sources and shipping logistics. Use of innovative PAT such as inline, real-time, flow-cytometry apoptosis assays in bioreactors at a range of volumes can facilitate process development and monitoring of batch-to-batch comparability. Currently, 20 regenerative medicine therapies have been approved. As has been seen with Dendreon’s Provenge experience, regulatory market approval does not guarantee commercial success. Adopting the approaches outlined herein, however, can mitigate risk.
References
1 Lanphier E. Program Introduction and Industry Update. Regenerative Medicine & Advanced Therapies State of the Industry Briefing. January 2016; http://alliancerm.org/event/regenerative-medicine-state-industry-briefing.
2 Hampson B, Ceccarelli J. Factories of the Future: Can Patient-Specific Cell Therapies Get There from Here? BioProcess Int. 14(4) 2016: S4–S7; www.bioprocessintl.com/wp-content/uploads/2016/04/14-4-sup-Hampson.pdf.
3 Lipsitz YY, Timmins NE, Zandstra PW. Quality Cell Therapy Manufacturing by Design. Nature Biotechnol. 34(4) 2016: 393–400; doi:10.1038/nbt.3525.
4 Joslin L, et al. Quality by Design: A CMO’s Perspective on Gaining Knowledge Faster and Better. Pharmaceut. Technol. February 2014 supplement; www.pharmtech.com/quality-design-cmos-perspective-gaining-knowledge-faster-and-better-0.
5 Szczypka Mark, et al. Single-Use Bioreactors and Microcarriers: Scalable Technology for Cell-Based Therapies. BioProcess Int. 12(3) 2014): S54–S68; www.bioprocessintl.com/wp-content/uploads/bpi-content/BPI_A_141203AR07_O_231760a.pdf.
6 Pralong A, et al. Paradigm Shift for Vaccine Manufacturing Facilities: The Next Generation of Flexible, Modular Facilities. Eng. Life Sci. 14(3) 2014): 244–53; doi:10.1002/elsc.201400027.
7 Hampson B. The Cost of Success Keeping Cost of Goods in Check for PatientSpecific Cell Therapies. Life Sci. Leader 12 May 2016; www.lifescienceleader.com/doc/the-cost-of-success-keeping-cost-of-goods-in-check-for-patient-specific-cell-therapies-0001.
8 Brindley DA, et al. Peak Serum: Implications of Serum Supply for Cell Therapy Manufacturing. Regen. Med. 7(1) 2012): 7–13; doi:10.2217/rme.11.112.
9 Stone E. Technological Advances Facilitate Autologous Cell Therapy Scale-Out. Gen. Eng. Biotechnol. News 25 August 2015; www.genengnews.com/insight-and-intelligence/technological-advances-facilitate-autologous-cell-therapy-scale-out/77900512/.
10 Kamani NR, Kumar U. Standardizing Practices for Cellular Therapy Manufacturing. BioPharm Int. 27(11) 2014; www.biopharminternational.com/standardizing-practices-cellular-therapy-manufacturing.
11 Bauer I, Spichiger S, Spichiger-Keller UE. Novel Single-Use Sensors for Online Measurement of Glucose. BioProcess Int. 10(8) 2012: 56–60; www.presens.de/fileadmin/user_upload/products/Press/2012_09_BPI_Novel-Single-Use-Sensors-for-Online-Measurement-of-Glucose_IBauer.pdf.
12 Logan D. The Introduction of the Futura Biomass Monitor for Monitoring Conventional and Disposable Bioreactors. Aber Instruments: Aberystwyth, UK, July 2010; www.il-biosystems.de/fileadmin/Produkt-PDFs/197_aber_futura_biomass_monitor_july2010.pdf.
13 Kuystermans Darrin, Avesh M, Al-Rubeai M. Online Flow Cytometry for Monitoring Apoptosis in Mammalian Cell Cultures As an Application for Process Analytical Technology. Cytotechnol. 68(3) 2016: 399–408; doi:10.1007/s10616-014-9791-3.
14 Hernandez R. Modular Manufacturing Platforms for Biologics. BioPharm Int. 28(5) 2015.
David Orchard-Webb, PhD, is a bioscience consultant currently serving as a research assistant for Professor Amine Kamen in the Department of Bioengineering at McGill University in Montreal, Quebec, Canada; biosci.resources@gmail.com; https://biosci-rc.com.