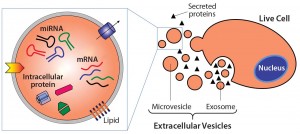
Figure 1: Extracelluar vesicles are essentially membrane-bound samples of cellular cytosol that transport cargoes such as miRNA, mRNA, and proteins. Exosomes form from fusion of multivesicular bodies with the plasma membrane, whereas microvesicles bud directly from live cells. Apoptotic bodies result from outward blebbing of the cell surface during apoptosis, but are not secreted from healthy cells.
Extracellular vesicles (EVs) are emerging as a potential alternative to some stem-cell–derived therapeutics (1, 2). Sometimes called exosomes, they are small, secreted vesicles that can possess similar therapeutic mechanisms to whole cells, possibly representing the active pharmaceutical ingredient. In the past 15 years, academic and industry interest in EVs has exponentially increased as mounting evidence demonstrates their role in physiology and pathology as well as their therapeutic potential.
In light of growing efforts in using EVs for research and therapy, optimizing EV manufacturing is important. However, many challenges come with their characterization, scalable manufacture, and regulatory status. Here, we briefly review the biology and therapeutic application of EVs, discuss associated challenges, and suggest how the biotechnology industry could play an important role in overcoming those challenges. Many cell manufacturing companies currently produce EVs but discard them as waste, thereby losing a potentially valuable resource with multiple purposes in a market that’s otherwise rich with an exorbitant cost of goods.
Definition and Uses
There are generally three types of EVs (Table 1): exosomes, microvesicles, and apoptotic bodies, as classified by their pathway of origin (3). However, this classification has seen wide adoption only recently, and ambiguity remains in the literature with overlap in characteristics between EV subsets. Essentially, these are membrane-bound samples of the cellular cytosol that transport various cargoes — e.g., miRNA, mRNA, and proteins — and can signal recipient cells to induce a range of responses (Figure 1).
Exosomes are formed intracellularly from inward budding of endosomal compartments. They are secreted when the resulting compartment (a multivesicular body) fuses with the cell membrane. Exosomes have largely been considered the most therapeutically interesting EV type. In practice, however, they are very difficult to separate from microvesicles that pinch directly from the cell membrane and apoptotic bodies that form during apoptosis (4). Given that current isolation methods cannot consistently separate the three EV subsets (and that no distinguishing markers have yet been identified for each) publications referencing the term exosomes often refer to a fraction of isolated vesicles within a particular density or size range, with unknown ratios of different vesicle types. Herein, therefore, we use the encompassing term extracellular vesicle.
EVs are thought to play a central role in the paracrine responses that therapeutic cells elicit in diseased or damaged tissue. This may explain the mechanism of action for positive therapeutic outcomes that cannot be explained by a persistence of transplanted cells. For example, EVs derived from mesenchymal stem cells (MSCs) have been able to reproduce similar therapeutic effects to MSCs in animal models for inflammatory diseases, autoimmune diseases, cancer, and acute tissue injuries (5, 6). EV therapy involves potential advantages and disadvantages (Table 2). EVs also could be used as vehicles for therapeutic delivery, for example across the blood–brain barrier (7).
Researchers are interested in EVs as mediators of paracrine signaling in both diseased and healthy tissues, particularly as transporters of RNA (miRNA and mRNA). EVs are thought to partake in normal physiology, including tissue repair, immune surveillance, suppression of inflammation, and neurotransmission (3). EVs are also known to play an important role in disease pathogenesis and facilitate tumor progression by inducing proliferation, angiogenesis, and immune suppression (3).
EVs As Byproducts of Cell Manufacturing
Given their potential as research tools and therapeutics, it is perhaps remarkable that many companies already produce usable EVs during cell production but discard them as waste (Figure 2). EVs are constantly secreted by cells, so producing them requires cell culture as does the manufacture of other biologics. However, unlike that of recombinant biopharmaceuticals, EV production does not require genetic manipulation of producer cells because all cells secrete them naturally. So cell manufacturers are producing EVs that are accumulating in conditioned media and simply disposed of. Cell types whose EVs have shown potential therapeutic benefit include MSCs (18), dendritic cells (19), cardiospheres (20) and neural stem cells (see the “Companies†box).
Companies Developing EV ProductsCapricor Inc. specializes in cell therapy for cardiac applications. Based on ReNeuron Group PLC uses neural stem cells (NSCs) to treat neurological and Systems Biosciences, Inc. (SBI) is a leader in developing technologies to isolate EVs Anosys Inc. started with the aim of manufacturing autologous dendritic-cell–derived |
Of the three companies we know to be currently developing EVs for commercial use (see the “Companies†box), two are producing them from primary cells that are being explored therapeutically, whereas the third produces EVs solely for research purposes. The only company that has attempted to start de novo EV therapeutic development (which would require simultaneous implementation of both cell growth and EV harvest processes) was acquired in 2005. Its status is currently unknown, perhaps reflecting greater logistical and operational challenges if cell manufacturing processes are not already in place.
Despite the apparently simple step for current cell manufacturers to integrate EV harvest into their processes, many companies have not done so. We postulate the most significant reasons to be a lack of standardized, scalable, reliable methods for EV isolation; and a lack of established criteria for an EV product that complies with current good manufacturing practices (CGMPs) (21).
A Need for Process Standardization
Cells constitutively secrete EVs into their media, making conditioned media the starting material for EV isolation. Presently, no standardized method exists for isolation and preparation of EVs from conditioned media, which is a challenge in moving the field forward. Harvesting EVs in serum-free media most likely will be required to ensure pure, safe, and regulatory-compliant products. The use of serum to supplement media can introduce EV contaminants because complete removal of serum EVs is challenging and would add steps to the manufacturing process. Assuming an appropriate medium is established, companies can choose from four principal methods of EV isolation (Figure 2): ultracentrifugation, precipitation, size-exclusion chromatography (SEC), and tangential-flow filtration (TFF). Each method has its own operational shortcomings and limits on scales of production (Table 3).
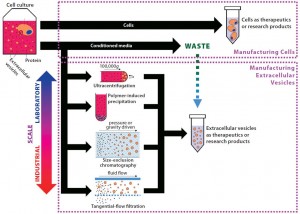
Figure 2: From byproduct to product — extracellular vesicle manufacturing; EVs are constitutively secreted by cells, and current isolation methods are presented herein. In most cell manufacturing processes, spent media are simply discarded. If cell manufacturers divert that effluent, adopt EV manufacturing processes, and scale up EV production, they could facilitate standardization in the field. EVs produced could be sold initially as research products, then potentially as therapeutics in the long term.
The most commonly used method for EV isolation, ultracentrifugation separates EVs based on size and density, unlike other methods that rely on size alone (24). In this process, first 2,000– 10,000g centrifugation removes media and large particles such as dead cells and cell debris. Ultracentrifugation at 100,000g then pellets EVs, largely separating them from remaining materials (25). However, this method is nonspecific to EVs; is prone to contamination with protein aggregates, genetic material, and particulates in the media (1, 26); and is complicated by the possibility of compacting EVs to form aggregates (27). Additionally, this process is time-consuming and highly labor- and energy-intensive. Large-scale ultracentrifugation is possible, having been used for production of other biologics, but it has not yet been evaluated for EVs.
Volume-excluding polymers can be used to precipitate EVs and allow their isolation through low-speed centrifugation. Several reagents are commercially available: e.g., ExoQuick (Systems Biosciences) and Total Exosome Isolation Reagent (Life Technologies). Precipitation is relatively straightforward and rapid; however, it suffers from a need to remove polymers from the final product as well as a lack of specificity to EVs (21). Other macromolecules (e.g., RNA–protein complexes) can coprecipitate with them.
Both SEC and TFF isolate EVs based on their size and can distinguish size more accurately than can ultracentrifugation. SEC has the unique advantage of enabling isolation in a single-step process. It was recently used to isolate EVs efficiently without coisolation of protein aggregates (28). SEC reagents are affordable, and the procedure is quick. However, its application to EVs is relatively new, and scalability is unexplored for this application. But SEC has been used successfully in the production of many biologics.
Nanomembrane ultrafiltration (29) and TFF (a similar method that uses ultrafiltration membranes) (30) can be used to concentrate EVs, and the latter is highly scalable. However, no ultrafiltration methods have yet been shown to be independently capable of purifying EVs. Instead they are used in conjunction with additional isolation methods to generate pure samples.
Both SEC and TFF have clear benefits and downsides. The commonly used ultracentrifugation currently serves as a benchmark for other isolation methods, but it has clear limitations. Precipitation-based methods are becoming increasingly popular due to their technical ease and speed; however, they suffer from lack of specificity. It seems likely that for larger-scale processing, using TFF for volume reduction and EV concentration must be followed by another technique to purify EVs (31). Still, significant process development is required to achieve scalable production methods.
That need for standardization and definition of best practices was emphasized in a position paper from the International Society for Extracellular Vesicles (ISEV), the world’s leading organization for EV researchers (27). To allow for comparability among results, standardization will be essential. Studies have shown that different isolation methods can yield EV preparations with morphological and quantitative differences, including different RNA profiles (24). Attempts at standardization are hampered not only by different isolation methods, but also by a lack of clarity in what actually constitutes an EV.
The Role That Industry Can Play
Industrial cell manufacturers are optimally placed to address the challenges we have outlined above, and in doing so they could generate profit from the sale or use of EVs. The route to bring a new EV therapy to market most likely will be long and complex. However, selling EVs as research products (as Systems Biosciences Inc. does) could rapidly generate revenue for cell manufacturers to support longer-term therapeutic development. Undeniably, the EV research field is growing quickly, and with that growth comes a need for EVs that can be used for research purposes, much like how recombinant proteins and primary cells were commercialized in the early history of the biotechnology industry. Currently, laboratory EV research relies on the inefficient and often costly and labor-intensive processes outlined above. If cell manufacturers can develop more efficient, cost-effective methods of EV production, then EV researchers surely will adopt their products.
Harvesting EVs requires minimal adjustment to a cell-culture process (Figure 2). The need for serum-free or EV-depleted media may be costly, but does not present an insurmountable barrier. In fact, it may become the standard for cell manufacturing if serum demand outstrips supply (32). Even so, many cell manufacturers continue to use serum-containing media. Serum-free media would not necessarily be required for the entire cell manufacturing process, however.
Instead, it could be used only in the final cell passage, where most cells and therefore EVs are produced, thus limiting potential concerns over the added expense of serum-free media use or its effect on final cell products. Additionally, use of serum-free media could reduce quality-control costs due to reduced probability of contamination.
The abundance of conditioned media from cell manufacturing provides a large volume of starting material with which to rapidly develop isolation processes and generate larger EV quantities than can be derived at laboratory scale. With greater EV quantities and availability of analytical tools and other resources, the ability to test and analyze consistency in EV preparations probably will be greater than what is possible on a laboratory bench. Such analyses — particularly if made available in databases including EVpedia and Vesiclepedia — may rapidly advance understanding of EV biology and help to address product and process standardization issues.
For therapeutic applications of EVs, addressing the issues outlined herein will be fundamentally important in testing comparability, lot-to-lot variability, and product drift, all of which are likely to be necessary for regulatory approval. Establishment of CGMP guidelines is one approach, and acceptable criteria for the following conditions may be useful:
- Size range for EVs
- Biochemical markers (for EVs broadly and for specific preparations)
- Purity of EV preparation and absence of undesired components
- Sterility of cell growth and EV isolation environments
- Optimal EV isolation methods (including for scalability, purity, and particular EV subsets)
- Development of EV stabilization methods
- Optimal storage conditions for EV stability
- Analytical methods and standards for all of the above
- Serum-free media use (likely to be compulsory) (32).
Those guidelines could inform manufacturers about quality control requirements and benefit the EV community more broadly. Establishing CGMP methods for EV manufacturing would provide acceptable criteria for EVs and EV processes in research, thereby driving standardization, bioprocess improvements, and ultimately increased regulatory confidence in EV products. Researchers could use commercially manufactured EVs as standards against which to compare their EV preparations, eventually leading to greater consensus in the field. In turn, those developments would drive and hopefully accelerate therapeutic development and eventually clinical adoption (33).
Worth the Trouble
As the biopharmaceutical field arose, researchers, manufacturers, and regulators acknowledged that fundamental differences distinguished more complex biological products and traditional small-molecule drugs. Those differences pervaded research, manufacturing, therapeutic applications, and regulations to greatly slow progress in a field that is now exceptionally important. As has been required for cell therapies, a step change in approach and process innovation also will be required for EVs.
Nobel Prize winner Joshua Lederberg noted that “as soon as you go into any biological process in any real detail, you discover it’s open-ended in terms of what needs to be found out about it†(34). Nowhere is that more applicable than to EV research, manufacture, and therapeutic application, where significant opportunity for discovery and understanding remains. Paradoxically, for cell manufacturers, it is exactly that need for progress in the EV field that might offset the risk of new process adoption. In a staged-pathway approach, EVs could be sold at research grade in the short term to fuel longer-term efforts. If the cell manufacturing industry adopts EV production and spearheads efforts toward standardization by establishing scalable processes and informing scientifically appropriate parameters, the entire field — from basic scientists to regulators and crucially to patients — could benefit.
Acknowledgments
We express our sincere thanks to the following companies and organizations that have contributed to the Centre for the Advancement of Sustainable Medical Innovation (CASMI) Translational Stem Cell Consortium (CTSCC) as funding and events partners, without whom the consortium and the benefits it will bring to stem-cell translation would be constrained: GE Healthcare, Centre for Commercialization of Regenerative Medicine (CCRM) (CCRM), Sartorius Stedim Biotech (formerly TAP Biosystems), Lonza, the California Institute for Regenerative Medicine (CIRM), the Strategies for Engineered Negligible Senescence (SENS) Research Foundation, UK Cell Therapy Catapult, the US NIH Center for Regenerative Medicine, the New York Stem Cell Foundation (NYSCF), ThermoFisher Scientific, Eisai, Medipost (US), Medipost (Korea), Celgene, Roche, and Oxford Biomedica. D.A. Brindley gratefully acknowledges personal funding from the Oxford Musculoskeletal National Institute for Health Research (NIHR) biomedical research unit, the Saïd Foundation, and the SENS Research Foundation. All authors also extend their gratitude to Felix-Reed Tsochas of the Saïd Business School (SBS), who provided invaluable support for this manuscript.
Disclosures
This publication represents the individual opinions of the authors and may not necessarily represent the viewpoints of their employers. D.A. Brindley is a stockholder in Translation Ventures Ltd., a company that among other services provides cell therapy biomanufacturing, regulatory, and financial advice to clients in the cell therapy sector. He is subject to the Chartered Financial Analyst (CFA) Institute’s codes, standards, and guidelines, so he must stress that this article is provided for academic interest only and must not be construed in any way as an investment recommendation. Additionally, at time of publication, Brindley and the organizations with which he is affiliated may or may not have agreed-upon and/or pending funding commitments from the organizations named herein.
References
1 Vishnubhatla I, et al. The Development of Stem Cell–Derived Exosomes As a Cell-Free Regenerative Medicine. J. Circ. Biomark. 143, 2014: 2.
2 Ratajczak MZ, et al. Pivotal Role of Paracrine Effects in Stem Cell Therapies in Regenerative Medicine: Can We Translate Stem Cell- Secreted Paracrine Factors and Microvesicles into Better Therapeutic Strategies? Leukemia 26, 2012: 1166–1173.
3 El Andaloussi S, et al. Extracellular Vesicles: Biology and Emerging Therapeutic Opportunities. Nat. Rev. Drug. Discov. 12, 2013: 347–357.
4 Kowal J, Tkach M, Théry C. Biogenesis and Secretion of Exosomes. Curr. Opin. Cell. Biol. 29, 2014: 116–125.
5 Robbins PD, Morelli AE. Regulation of Immune Responses By Extracellular Vesicles. Nat. Rev. Immunol. 14, 2014: 195–208.
6 Lamichhane TN, et al. Emerging Roles for Extracellular Vesicles in Tissue Engineering and Regenerative Medicine. Tissue Eng. Part B Rev. 21(1) 2015: 45–54.
7 Alvarez-Erviti L, et al. Delivery of siRNA to the Mouse Brain By Systemic Injection of Targeted Exosomes. Nat. Biotechnol. 29, 2011: 341– 345.
8 Davies BM, et al. Repairing Damaged Tendon and Muscle: Are Mesenchymal Stem Cells and Scaffolds the Answer? Regen. Med. 8, 2013: 613–630.
9 Linero I, Chaparro O. Paracrine Effect of Mesenchymal Stem Cells Derived from Human Adipose Tissue in Bone Regeneration. PLoS ONE 9(9) 2014: e107001.
10 Karp JM, Leng Teo GS. Mesenchymal Stem Cell Homing: The Devil Is in the Details. Cell Stem Cell 4, 2009: 206–216.
11 Van Buskirk RG, et al. Hypothermic Storage and Cryopreservation. BioProcess Int. 2(10) 2004: 42–49.
12 Lamparski HG, et al. Production and Characterization of Clinical Grade Exosomes Derived from Dendritic Cells. J. Immunol. Meth. 270, 2002: 211–226.
13 O’Cearbhaill ED, Ng KS, Karp JM. Emerging Medical Devices for Minimally Invasive Cell Therapy. Mayo Clin. Proc. 89, 2014: 259– 273.
14 Brindley D, et al. Bioprocess Forces and Their Impact on Cell Behavior: Implications for Bone Regeneration Therapy. J. Tiss. Eng. 2(1) 2011: 2011_620247.
15 Kircher MF, Gambhir SS, Grimm J. Noninvasive Cell-Tracking Methods. Nat. Rev. Clin. Oncol. 8, 2011: 677–688.
16 Nguyen PK, Riegler J, Wu JC. Stem Cell Imaging: From Bench to Bedside. Cell Stem Cell 14, 2014: 431–444.
17 Xu X, Vugmeyster Y. Challenges and Opportunities in Absorption, Distribution, Metabolism, and Excretion Studies of Therapeutic Biologics. AAPS J. 14, 2012: 781–791.
18 Bruno S, et al. Mesenchymal Stem Cell–Derived Microvesicles Protect Against Acute Tubular Injury. J. Am. Soc. Nephrol. 20, 2009: 1053–1067.
19 Morse MA, et al. A Phase I Study of Dexosome Immunotherapy in Patients with Advanced Non-Small Cell Lung Cancer. J. Transl. Med. 3(1) 2005: 9.
20 Ibrahim AG-E, Cheng K, Marbán E. Exosomes As Critical Agents of Cardiac Regeneration Triggered By Cell Therapy. Stem Cell Rep. 2, 2014: 606–619.
21 Lötvall J, et al. Minimal Experimental Requirements for Definition of Extracellular Vesicles and Their Functions: A Position Statement from the International Society for Extracellular Vesicles. J. Extracell. Vesicles 3, 2014: 26913.
22 Patel S, et al. An Analysis of Variability in the Manufacturing of Dexosomes: Implications for Development of an Autologous Therapy. Biotechnol. Bioeng. 92, 2005: 238–249.
23 Escudier B, et al. Vaccination of Metastatic Melanoma Patients with Autologous Dendritic Cell (DC) Derived- Exosomes: Results of the First Phase I Clinical Trial. J. Transl. Med. 3, 2005: 10.
24 Van Deun J, et al. The Impact of Disparate Isolation Methods for Extracellular Vesicles on Downstream RNA Profiling. J. Extracell. Vesicles 3, 2014: www.ncbi.nlm.nih.gov/pmc/articles/PMC4169610.
25 Théry C. Exosomes: Secreted Vesicles and Intercellular Communications. F1000 Biol. Rep. 3, 2011: 15.
26 Vlassov AV, et a;. Exosomes: Current Knowledge of Their Composition, Biological Functions, and Diagnostic and Therapeutic Potentials. Biochim. Biophys. Acta BBA — Gen. Subj. 1820, 2012: 940–948.
27 Witwer KW, et al. Standardization of Sample Collection, Isolation and Analysis Methods in Extracellular Vesicle Research. J. Extracell. Vesicles 2, 2013: 20360.
28 Böing AN, et al. Single-Step Isolation of Extracellular Vesicles By Size-Exclusion Chromatography. J. Extracell. Vesicles 3, 2014: 23430.
29 Cheruvanky A, et al. Rapid Isolation of Urinary Exosomal Biomarkers Using a Nanomembrane Ultrafiltration Concentrator. Am. J. Physiol. Renal Physiol. 292, 2007: F1657–1661.
30 Kim H-S, et al. Proteomic Analysis of Microvesicles Derived from Human Mesenchymal Stem Cells. J. Proteome Res. 11, 2011: 839–849.
31 Nordin JZ, et al. Ultrafiltration with Size-Exclusion Liquid Chromatography for High Yield Isolation of Extracellular Vesicles Preserving Intact Biophysical and Functional Properties. Nanomed. Nanotechnol. Biol. Med. 4 February 2015.
32 Brindley DA, et al. Peak Serum: Implications of Serum Supply for Cell Therapy Manufacturing. Regen. Med. 7, 2012: 7–13.
33 Davies BM, et al. Quantitative Assessment of Barriers to the Clinical Development and Adoption of Cellular Therapies: A Pilot Study. J. Tissue. Eng. 5, 2014: 2041731414551764.
34 Geigert J. Biologics Are Not Chemical Drugs. Chall. CMC Regul. Compliance Biopharm. Biol. 2013: 21–33. •
James A. Smith (researcher at the Oxford- UCL Centre for Advancement of Sustainable Innovation’s Translational Stem Cell Consortium) and Kelvin S. Ng (graduate research assistant in the Harvard–MIT Health Sciences and Technology program) contributed equally to this work. Benjamin E. Mead is a graduate research assistant in the Harvard–MIT Health Sciences and Technology program; Sue Dopson is the Rhodes Trust Professor of Organisational Behaviour and associate dean of faculty at Saïd Business School; Brock Reeve is executive director of the Harvard Stem Cell Institute and director of the Poliwogg Regenerative Medicine Fund; James Edwards is a Marie Curie Fellow and associate professor in the University of Oxford’s Nuffield Department of Orthopedics, Rheumatology, and Musculoskeletal Sciences; Matthew J.A. Wood is a professor of neuroscience in the University of Oxford’s department of physiology, anatomy, and genetics; Andrew J. Carr is the Nuffield Professor of Orthopedic Surgery and director of the University of Oxford’s Nuffield Department of Orthopedics, Rheumatology, and Musculoskeletal Sciences (NDORMS); and Kim Bure is director of regenerative medicine at Sartorius Stedim Biotech.
Joint senior authors Jeffrey M. Karp (associate professor of medicine at Brigham & Women’s Hospital and at Harvard Medical School, jmkarp@partners.org) and David A. Brindley (Cooksey– Botnar–Saïd Fellow in healthcare translation at the University of Oxford’s NDORMS and the Saïd Business School, research fellow at the Harvard Stem Cell Institute, senior research fellow at the University College London’s Centre for Behavioural Medicine, and a member of the Stanford–UCSF FDA Centre for Regulatory Science and Innovation, david.brindley@ndorms.ox.ac.uk) are also corresponding authors.
Hi there,
has there been some recent publications on what a GMP production and isolation/purification process for EVs (in particular exosomes) would look like? … and accepted by relevant regulatory agencies?
thanks for a brief feedback!
franco